Ti ricordo sempre di rimanere cosi come ti sei sempre mostrato "uomo di scienza, ma sopratutto umano"
Giovanni Puccio, Ricercatore e Uomo di Scienza.
E' un gran privilegio aver vissuto una vita difficile. Ci sono cose più straordinarie di altre e io sono una di queste.
EUROFLAG TODAY
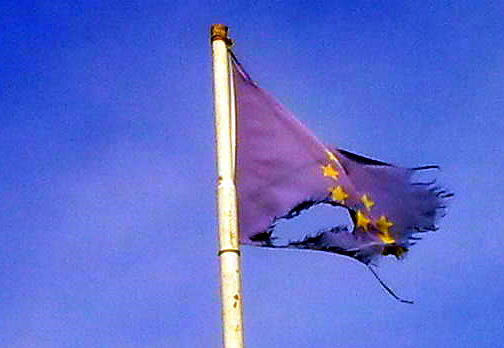
mercoledì 28 marzo 2012
martedì 27 marzo 2012
Oxidative Stress in Heart Failure: Current Understanding and Prospective
Oxidative Stress in Heart Failure: Current
Understanding and Prospective
OSxinidgaatli,v KehSatpreers,s PaanldacHe,eaanrtd FKauilmuraer
Pawan K. Singal, Neelam Khaper, Vince Palace,
and Dinender Kumar
Institute of Cardiovascular Sciences, St. Boniface General
Hospital Research Centre and Department of Physiology,
Faculty of Medicine, University of Manitoba, Winnipeg. Canada
Abstract. Our knowledge of the pathophysiology of heart
failure has advanced far beyond the classic concept of the
hemodynamic overload model and associated neurohumoral
changes. Current interest lies in understanding the fundamentals
of cellular defects to identify new molecular targets
for therapies. Some of the new target sites being explored
are in_ammatory cytokines, nitric oxide, oxidative
stress and apoptosis during the remodelling processes such
as cardiac hypertrophy and dilation. The present review
provides an overview of the chemistry/biochemistry of free
radicals as well as a discussion of some defense mechanisms
that have evolved and adapted to combat these toxic oxygen
species. The probable role of oxidative stress in the
pathogenesis of heart failure both from animal data and
heart failure patients is also presented.
Key Words. oxygen radicals, antioxidants, cardiac dysfunction
The Oxygen Story
Although we have witnessed a profound development
in the understanding of oxygen toxicity in heart disease
in the past three decades, the story of oxygen
toxicity started more than two centuries ago [1]. It was
Lavoisier, in 1785, who made the ~rst observation that
oxygen has two main effects, i.e. it supports life but it
also has toxic side effects [2]. More than a century later,
in 1899, Lorrain Smith demonstrated that increased
oxygen tension results in lung congestion in mice, rats
and pig [3]. At the beginning of the twentieth century,
Gomberg demonstrated the presence of triphenylmethyl
molecule as a radical species in organic chemistry
[4]. Subsequently, it was theorized that the damaging
effects of O2 were due to the formation of the
superoxide anion [5].
The pioneering work of McCord and Fridovich regarding
the discovery of superoxide dismutase [6], inspired
biologists and clinicians to study the role of free
radicals in biology and medicine. Thus, a large body
of evidence has accumulated showing that biological
systems are capable of producing a variety of reactive
oxidants which play an important role in various human
diseases [7,8]. Animal studies on catecholamineinduced
cardiomyopathy [9], adriamycin-induced
cardiomyopathy [10] and ischemia-reperfusion injury
[11] have now provided solid evidence for the role of
free radicals in the mediation of cardiac injury [1,12].
The present review provides some basic information
on free radicals and antioxidants and their signi~cance
in the pathogenesis of heart failure.
Oxygen Free Radicals
Free radicals are highly reactive atoms or molecules
with an unpaired electron in their outer orbits. The
production of free radicals occurs either by the addition
or by the removal of an electron in a reduction/
oxidation reaction. Since oxygen has two electrons
with parallel spin in its outermost shell, it is
characterized as a diradical which requires four electrons
to be completely reduced to water [13–15]. Oxygen
is also the terminal acceptor of electrons for oxidative
phosphorylation and this tetravalent reduction
is associated with the production of high energy phosphates
(Fig. 1). However, sequential univalent reduction
results in the formation of reactive oxygen intermediates
[7,8,13–15]. In the univalent reduction
pathway, the addition of a single electron to molecular
oxygen results in the production of superoxide anion
radical (O2
2?). The addition of another electron to the
O2
2? results in the formation of peroxide anion which
protonates to form hydrogen peroxide (H2O2). The latter
is not a radical by itself, but is capable of causing
cell damage by interacting with transition metals such
as iron. A single electron reduction of H2O2 results in
the formation of the hydroxyl radical (?OH) which is
highly reactive, has an extremely short half-life, and
therefore has a very limited diffusion capacity [13–15].
The addition of a fourth electron ~nally results in the
formation of water. The ~rst excited state of O2 is a
singlet oxygen (1O2) which can also initiate oxygen
Address for correspondence: Dr. Pawan K. Singal, Institute of
Cardiovascular Sciences, St. Boniface General Hospital Research
Centre, 351 Tache Avenue, R3022,Winnipeg, MB R2H 2A6, Canada.
Email: psingal@sbrc.umanitoba.ca
111
Heart Failure Reviews 1999;4:111–120
© Kluwer Academic Publishers. Boston. Printed in U.S.A.
radical chain reactions [13,14]. This univalent electron
reduction reaction is illustrated in ~gure 1.
Reactive oxygen intermediates such as O2
2?, H2O2,
?OH and 1O2 are called activated oxygen species and are
collectively known as partially reduced forms of oxygen
(PRFO) [13]. Superoxide radical reacts with nitric
oxide during reperfusion to form peroxynitrite which
also has a harmful effect by opposing the vasodilatory
effect of nitric oxide [16]. These reactive species can
interact with macromolecules and initiate free radical
chain reactions resulting in membrane and cell damage
[13–15,17].
Free Radical Mediated Cell Injury
The targets of free radical attack are the cell membranes
and subcellular organelles. The lipid peroxidation
chain reaction is initiated by the removal of a
hydrogen atom from the unsaturated site in a fatty
acid resulting in the production of a lipid radical. This
radical can further react with other neighbouring
polyunsaturated fatty acids (PUFA) to propagate the
reaction [13,18]. The addition of an oxygen molecule
to these lipid radicals results in the formation of lipid
peroxides. Free radical-induced lipid peroxidation has
been suggested to alter membrane structure and function
[13–15]. Considerable evidence also suggests that
PRFO can modify protein structure and function ultimately
affecting cell metabolism. In this regard, proteins
rich in sulphydryl groups are highly susceptible
to free radical attack [13]. In the myocardium, oxygen
radicals have been shown to effect Na1/Ca11 exchange,
Na1-K1 ATPase and Ca11 ATPase activities
[19–22]. Free radicals can also attack the nucleic acids
by producing base damage, single strand breaks, adducts
and chromosomal aberrations. Such modi~cations
have been shown to cause cellular abnormalities
such as mutations and cell death [12–15].
Fig. 1. A diagramatic representation of the production of oxygen free radicals by univalent reduction pathway. Different antioxidants
(enzymatic and non-enzymatic) in the biological system may offer protection against free radical-induced injury.
112 Singal, Khaper, Palace, and Kumar
The Antioxidant Defense System in
the Heart
Under normal physiological conditions, the tissue concentration
of free radicals is maintained by a system of
enzymatic and non-enzymatic antioxidants that have
evolved and been conserved during the evolution of
aerobic life and constitute “antioxidant reserve” in the
heart [17].
Enzymatic Antioxidants
Superoxide dismutase (SOD)
This enzyme is the ~rst line of defense against free radical
attack. There are several different types ofSODthat
differ based on the metallic prosthetic group attached
within the protein structure. The most common forms
are CuZnSOD (molecular weight 32,000) which is present
in the cytoplasm and MnSOD (molecular weight
80,000) found in the mitochondria. Superoxide dismutase
activity has been reported to be signi~cantly less in
the heart than in the liver [23–25]. It was the discovery
of SOD which led to the realization that O2
2? is formed in
vivo in living organisms [6]. Two superoxide anions can
react together to produce a spontaneous dismutation,
however, this reaction is much faster in the presence of
SOD (23109M21 sec21) [13,23–25].
Glutathione peroxidase
This selenium-dependent enzyme (molecular weight
~84,000), which is present mainly in the cytoplasm, represents
the second line of defense against free radicalmediated
damage. It catalyzes the reduction of hydrogen
peroxides using glutathione (GSH) as a source of
reducing power and forming oxidized glutathione
(GSSG), water and/or alcohol. This enzyme is present in
relatively high concentrations in the human heart
[13,23,25] and is therefore considered an important antioxidant
enzyme in that organ. A non selenium-dependent
form of GSHPx, which is non-speci~c to H2O2, but
which dismutates organic peroxides is also present [26].
Catalase
This enzyme (molecular weight 240,000) is also very
important in the metabolism of hydrogen peroxide produced
by SOD. Present at relatively low concentrations
in the heart, it converts H2O2 to water and oxygen.
However, the difference between catalase and
glutathione peroxidase is that GSHPx is more effective
at low concentrations of H2O2, i.e., in lM range,
whereas catalase is more effective at the mM concentrations
of H2O2 [13,15,27].
Non-enzymatic antioxidants
Non-enzymatic antioxidants include vitamins such as
tocopherols, ascorbate and carotenes as well as other
biological molecules including glutathione, uric acid
and metal binding proteins.
Vitamin E
Vitamin E functions as one of the major antioxidant
defense systems in our body. Vitamin E is a generic
term that includes a group of eight structurally related
compounds. These are further sub-divided into two
groups: tocopherols and trienols. Of these, d-a-tocopherol
is the most common type of vitamin E absorbed
from the human diet [28]. It is a strong biological antioxidant.
Because of its lipophillicity, vitamin E offers
maximum protection against free radical attack in cellular
and subcellular membranes. it reacts with free
radicals, yielding lipid hydroperoxides which can be
removed by the GSHPx enzyme system. In this reaction,
vitamin E donates a hydrogen atom, resulting in
the generation of a hydroperoxide and vitamin E radical.
This effectively terminates lipid peroxide-mediated
chain reaction and is therefore called a “chain
breaking antioxidant” [1,13,28–30]. Vitamin E also
functions synergistically with ascorbic acid to terminate
free radical chain reactions. Recent studies have
reported a new role for vitamin E that is independent
of its antioxidant property. These include inhibition of
smooth muscle cell (SMC) proliferation and growth
during atherosclerosis, occurring mainly as a result of
its ability to activate the release of TGF-b [31].
Vitamin C
Vitamin C is a water-soluble molecule which quenches
reactive oxygen metabolites directly, with the resultant
formation of dehydroascorbate. Vitamin C is also
thought to play an important role in the regeneration
of a tocopherol. It is present in micromolar concentrations
in the cytoplasm as well as in serum [13,32].
b carotene
b carotene, a precursor of vitamin A, is known to
quench active oxygen species. These reactions operate
maximally at low-oxygen tension as compared to vitamin
E which reacts at high oxygen tension [33].
Epidemiological studies have shown that increased
levels of b carotene and other carotenoids are associated
with a decreased risk of cardiovascular diseases
[34,35].
Glutathione
Glutathione is a tripeptide which is present in high
concentrations in the majority of the eukaryotic cells.
One of its important functions is to protect cells
against peroxides generated during aerobic metabolism.
It undergoes redox cycling between the reduced
(GSH) and oxidized (GSSG) forms [13,36,37]. In the
heart, glutathione is predominantly (.95%) in the
GSH form. It also acts as a cosubstrate for GSHPx
and plays an important role in defending against free
radical-mediated lipid peroxidation. This results in the
Oxidative Stress and Heart Failure 113
increased formation of GSSG. Depletion of GSH to
20–30% of its normal concentration renders the cell
susceptible to free radical attack [38,39]. The redox
ratio, which is the ratio of reduced to oxidized glutathione
(GSH/GSSG) is used as a sensitive index of
oxidative stress. Speci~cally, an increase in the redox
ratio indicates reduced oxidative stress, and vice versa
[1,13–15].
Other Biological Molecules
Uric acid, a waste product of purine metabolism, has
been shown to directly interact with ?OH and prevent
oxidation [40], suggesting that there is antioxidant potential
for this molecule in biological systems [13]. Iron
chelators, such as desferrioxamine, inhibit lipid peroxidation
and reduces the injury associated with ischemia-
reperfusion [41,42]. Ubiquinone also acts as a
potent inhibitor of lipid peroxidation by directly
quenching free radicals [43]. Various metal binding
proteins such as ferritin, transferrin, metallothioneins
and ceruloplasmin are also known to inhibit lipid peroxidation
[13,44].
Methods of Measuring Free
Radical-Mediated Damage
Free radical-mediated damage includes oxidative
modi~cation of cellular proteins, lipids and nucleic acids.
Lipid peroxidation is a free radical-mediated process,
which involves the formation of lipid peroxides
within cell membranes and organelles [13,44,45]. Lipid
peroxidation is detected through the estimation of
malondialdehyde (MDA) either by UV light, HPLC or
the thiobarbituric acid (TBA) method [13,45,46]. Direct
detection of free radicals is done by ESR spectroscopy,
although with this technique it is not possible to detect
low concentrations of free radicals [13]. Other methods
of assessing oxidative stress include determination of
diene conjugates, measurement of gases such as ethane,
pentane and isoprotanes in exhaled air, measuring
the redox ratio and the content and activity of endogenous
antioxidants and quantifying lipid hydroperoxides.
Although the MDA assay has been shown to lack
speci~city, it may detect saturated and unsaturated
aldehydes as well as other non lipid-related substances
[46], when used in conjunction with studies of the redox
ratio as well as antioxidant activity, it provides meaningful
information [1,12].
Oxidative Stress and Antioxidants in
Heart Failure
The classic de~nition of oxidative stress includes “a disturbance
in the prooxidant and antioxidant balance, in
favour of the former” [47]. The most common pathological
conditions that are attributed to oxidative stress
mediated damage are atherosclerosis, diabetes, cancer,
arrhythmia, rheumatoid arthritis, in_ammatory bowel
disease, and several neurodegenerative diseases. In
fact, this list of pathological conditions related to oxidative
stress continues to grow. In recent years, substantial
evidence has accumulated from acute and chronic
studies to suggest the role of antioxidants and oxidative
stress in the pathogenesis of heart dysfunction and
failure both in animal studies and from clinical trials
[12,13,25]. Early studies on catecholamine-induced
cardiomyopathy [9,18], adriamycin-cardiomyopathy
[10] and ischemia-reperfusion injury [11] provided the
basis for further investigation on understanding free
radical involvement in cardiac injury [1,12,13].
Acute Studies
Ischemia-reperfusion (I/R) is one of the most extensively
studied models of acute heart failure. Increased
free radical production and depressed antioxidant reserve
has been documented to have serious functional
consequences after I/R. Many studies have reported
the production of free radicals in reperfused hearts in
animals [25,48,49] and humans [50,51]. Studies on isolated
perfused rat hearts showed increased production
of H2O2 during ischemia as well as early reperfusion
[52]. An inverse correlation between oxidative stress
and cardiac function has also been documented in patients
who were subjected to global ischemia and
reperfusion during coronary artery bypass graft surgery
[53]. In isolated perfused rabbit hearts, a decline
in developed force during ischemia was accompanied
by a progressive decline in tissue glutathione content
and in the redox ratio indicating increased oxidative
stress [23]. A decrease in non-enzymatic antioxidants
such as tocopherol, retinol and ascorbic acid and redox
ratio and an increase in lipid peroxides has also recently
been reported in rat hearts subjected to 45 min
of ischemia and 15 min of reperfusion [54]. A reduction
in infarct size by SOD and catalase administration was
also reported in dogs that underwent 90 min of ischemia
and 24 hrs of reperfusion [55]. Similarly, another
study using transgenic mice, which overexpressed
MnSOD, demonstrated improved cardiac
function and decreased release of lactate dehydrogenase
in mice hearts subjected to ischemia-reperfusion
[56]. Lack of protection with antioxidants has also
been reported in some studies. SOD was found ineffective
in modulating ischemia reperfusion-induced
changes in pig hearts [57].
There is now overwhelming evidence that oxidative
stress also plays a major role in myocardial stunning
[11]. It has recently been suggested that approximately
80–85% of stunning can be attributed to free
radicals and the rest (15–20%) is due to Ca11 overload
[58]. Antioxidant therapy suppressed the production of
free radicals and attenuated myocardial stunning, suggesting
a cause and effect relationship [11,59]. An ele-
114 Singal, Khaper, Palace, and Kumar
gant study by Li and colleagues, using adenovirus-mediated
gene transfer technique to introduce SOD in the
rabbits, demonstrated protection against myocardial
stunning [60].
Chronic Heart Failure
Catecholamine-induced cardiomyopathy
Increased plasma levels and decreased tissue levels of
catecholamines have been reported in acute MI and
heart failure conditions [61]. Excess catecholamines
have been shown to cause arrhythmias as well as
cardiomyopathy [9]. Production of free radicals upon
autooxidation of catecholamines play a critical role in
cardiomyopathy [9,18]. A recent study reported increased
lipid peroxidation in isoproterenol administered
rat hearts [62]. Pretreatment of rats with vitamin
E reduced catecholamine-induced arrhythmia and
other functional changes [63]. Conversely, animals fed
a vitamin E de~cient diet were found to be more susceptible
to adriamycin- [64] and catecholamine-induced
cardiomyopathic changes [18].
Adriamycin Cardiomyopathy
It is now well established that adriamycin
cardiomyopathy is associated with increased free radicals
and decreased antioxidant status in the heart
[65–68]. Direct evidence for free radical involvement
was provided by studies which showed that vitamin
E-treated mice were more resistant to adriamycin
cardiotoxicity [69]. Moreover, rats maintained on a vitamin
E de~cient diet were more susceptible to
adriamycin cardiotoxicity [10,64]. Treatment with
probucol, an antioxidant as well as a lipid-lowering
drug, modulated the pathogenesis of heart failure due
to adriamycin [66,67].
Diabetic Cardiomyopathy
Evidence is available to suggest the role of increased
oxidative stress and depressed antioxidant enzyme activities
in the pathogenesis of diabetic cardiomyopathy.
A decrease in myocardial SOD and catalase activity
and an increase in oxidative stress has been demonstrated
in STZ-induced diabetic rats [70]. Furthermore,
probucol treatment in these rats resulted in improved
cardiac function [70]. Similarly, some other
studies reported reduced oxidative stress upon vitamin
E supplementation in animals and patients [71–73].
Hypertrophy and Heart Failure
An increase in endogenous antioxidant enzyme activities
and a decrease in lipid peroxidation have been
reported in the hypertrophy stage induced by chronic
pressure overload in rats [74,75] and guinea pigs
[76,77]. Myocytes isolated from hypertrophied rat
hearts also showed increased antioxidants and reduced
lipid peroxidation [78]. The heart failure stage in
guinea pigs was characterized by depressed cardiac
function, dyspnea, as well as lung and liver congestion.
At this stage, a signi~cant decline in myocardial SOD
and GSHPx activities and a decrease in the redox ratio
were indicative of increased oxidative stress [76]. Antioxidant
treatment of the guinea pigs with vitamin E
decreased oxidative stress and delayed the occurrence
of heart failure [77]. In a chronic volume overload
model in dogs, decrease in contractility was associated
with an increase in MDA and a decrease in antioxidant
enzyme activity. Vitamin E treatment modulated some
of these changes [79].
Myocardial Infarction and
Congestive Heart Failure
Recent data from our laboratory have demonstrated
the role of oxidative stress in the pathogenesis of heart
failure following myocardial infarction (MI) [1,12].
Changes in myocardial antioxidants as well as oxidative
stress have been described in the surviving myocardium
of rats subjected to MI. These changes correlated
with cardiac function at different stages of failure
[80]. In this study, maintenance of hemodynamic function
in early stages (non-failure stage) was accompanied
by a signi~cant decrease in oxidative stress and
lipid peroxidation while the antioxidant reserve was
maintained. In late stages, where hemodynamic function
was depressed, the myocardial antioxidants
GSHPx, catalase, SOD and vitamin E were also
signi~cantly decreased while oxidative stress was increased
[80]. In addition to the myocardium, the levels
of vitamin E and A were also depleted in the storage
organs liver and kidney in rats with severe heart failure
[81]. Supplementation of these rats with vitamin E
before subjecting them to coronary artery ligation surgery
modulated these changes [81].
In a study of regional changes in the two ventricles
during the sequelae of heart failure, the antioxidant
de~cit and an increase in oxidative stress occurred ~rst
in the left ventricle [80]. In moderate to severe heart
failure stages, these changes also occurred in the right
ventricle [82]. Thus, oxidative stress and antioxidant
changes correlated with depressed cardiac function in
the respective ventricles. In another study, using the
same animal model, we reported that improved cardiac
function after treatment with the afterload reducing
drugs captopril or prazosin was related to the maintenance
of myocardial endogenous antioxidant status and
decrease in oxidative stress [83,84].
Antioxidant vitamins such as vitamin C, carotenoids
and vitamin E have been shown to decrease lipid peroxidation
and reduce atherogenesis and the risk of
coronary heart disease. Pretreatment with vitamin E
limited myocardial necrosis [85–87] while combined
Oxidative Stress and Heart Failure 115
pretreatment with vitamins E and C in ischemia-reperfusion
settings was found to protect the myocardium
and reduce the infarct size in pigs [88]. On the other
hand, pigs supplemented with oral vitamin E did not
show reduction in infarct size after ischemia-reperfusion
[89].
Clinical Studies
An increase in oxidative stress has recently also been
documented in heart failure patients. Malondialdehyde
levels were found to be signi~cantly higher in congestive
heart failure patients [90–92]. Increased breath
pentane (a byproduct of lipid peroxidation) levels were
reported in exhaled air of CHF patients and treatment
with captopril attenuated this rise and improved the
patient’s clinical condition [93,94]. Furthermore, it has
also been demonstrated that the increase in lipid peroxidation
correlates with the severity of heart failure
[92,95,96]. In addition to an increase in free radical
activity, a decrease in enzymatic and non-enzymatic
antioxidants has also been reported in patients with
congestive heart failure [90–92,96]. In another recent
study, Yucel and colleagues reported a signi~cant decrease
in blood glutathione and erythrocyte SOD activity
and an increase in lipid peroxidation in dilated
cardiomyopathic heart failure patients [97]. All of these
clinical studies provided strong support for the involvement
of oxidative stress in the pathogenesis of
heart failure.
Various clinical trials have examined the bene~cial effects
of antioxidant vitamins in MI and heart failure
conditions. The Health Professional Follow-up Study
[98] and the Nurses Health Study [99] found a decrease
in the incidence of coronary artery disease in men and
women supplemented with vitamin E. In another
study, a cocktail containing antioxidant vitamins A, C,
E and b-carotene resulted in a decrease in oxidative
stress as well as the infarct size in MI patients [100].
The Cambridge Heart Antioxidant Study (CHAOS)
reported a substantial decrease in the incidence of MI
in patients receiving vitamin E [101]. On the other
hand, some studies found no correlation between serum
alpha-tocopherol concentration and subsequent
episodes of MI on cardiovascular disease related death
[102,103].
Oxidative Stress, Apoptosis and
Heart Failure
Although the molecular mechanisms involved in initiating
oxidative stress are not known, some recent
studies have suggested the role of transcription factors
such as NFjB and cytokines in linking oxidative stress
to remodelling processes, such as apoptosis (Fig. 2).
Findings from several in vitro studies and animal models
suggest that apoptosis occurs in response to ischemia-
reperfusion, myocardial infarction, and chronic
pressure overload [104–107], all of which are conditions
that generate oxidative stress [12,13]. More recently,
the loss of myocytes through apoptosis or programmed
cell death, has been reported in the infarct regions of
myocardium from MI patients [108] as well as in patients
with end-stage heart failure [109,110]. The importance
of oxidative stress in apoptosis is also
con~rmed by the observation that knock-out mice lacking
MnSOD die early due to cardiomyopathy as compared
to normal mice [111]. Mechanistic investigations
of apoptosis suggest that tumor suppression protein
p53 triggers and Bcl2 inhibits the process in cardiomyocytes
[107,112]. The mechanism of action of Bcl2 for the
prevention of apoptosis has also been suggested to be
mediated by an antioxidant pathway whereby Bcl2
gene product interacts with MnSOD and inhibits apoptosis
[113]. Cytokines as potent inducers of apoptosis
have also recently been suggested in CHF conditions
[114]. Although the role of oxidative stress in apoptosis
and heart failure patients have been documented, the
exact contribution of apoptosis in heart failure still
remains to be established. Some studies suggest that
Fig. 2. Events linking oxidative stress and heart failure in
cardiac stress conditions.
116 Singal, Khaper, Palace, and Kumar
apoptosis contributes to only 0.2% of the total
cardiomyocyte death at any given time [110]. Furthermore,
the underlying molecular mechanisms that regulate
this remodelling event are still not fully understood.
However, more details are provided in other
papers in this focussed issue.
Cytokines and Oxidative Stress in
Heart Failure
Increasing evidence from both animal [115] and human
studies (116) have reported elevated levels of cytokines
such as tumor necrosis factor a (TNF-a), interleukin-
1a (IL-1a) and atrial naturietic factor in MI
and failure conditions (Fig. 2). Increased concentrations
of these cytokines were shown to have a direct
relation to the severity of failure [25,116,117]. Recent
studies suggest that the deleterious effect of TNF-a
is receptor-mediated and it also directly activates nitric
oxide, which is cytotoxic to myocardial cells by
virtue of its ability to produce free radicals and cause
apoptosis [118] (Fig. 2). The pathological potential of
TNF-a is demonstrated in a study where transgenic
mice overexpressing TNF-a in the heart developed
cardiomyopathy associated with apoptosis [119]. In a
recent study, it was demonstrated that TNF-a and
Ang II induce hypertrophy in cultured neonatal cardiac
myocytes by virtue of producing free radicals and
antioxidants such as BHA, vitamin E and catalase inhibited
this effect of TNF-a and Ang II by scavenging
the radicals [120]. Another new concept suggests that
TNF-a not only produces PRFO, but is also activated
by hydrogen peroxide, in the presence of a P38MAP
kinase [121]. Further studies are required to clarify
this point. A detailed discussion on these aspects is
presented in other articles in this focussed issue of
the journal.
Conclusions
Although there is convincing evidence from both animal
studies and patients that oxidative stress plays a
very important role in the pathogenesis of heart failure
there are not enough clinical trials which have assessed
the use of antioxidant therapy in the treatment of
heart failure. The laboratory ~ndings described here
suggest newer target sites for the prevention of heart
failure as well as better management of patients by
using appropriate antioxidant therapies.
Acknowledgments
Research reported here was done with grant support from the
Medical Research Council of Canada and the Heart and Stroke
Foundation of Manitoba. Dr. Singal is supported by a career
award from the Medical Research Council of Canada, Dr. Palace
by a fellowship from the Manitoba Health Research Council and
Ms. Khaper by the Heart and Stroke Foundation of Canada.
References
1. Singal PK, Khaper N, Palace V, Kumar D. The role of oxidative
stress in the genesis of heart disease. Cardiovasc Res
1998;40:426–432.
2. Lavoisier A-L. Alterations qu’eprouve 1’air resire. Recueil
des memoires de Lavoisier. 1785, Read to the Societe de
Medicine. Reprinted as part of “Memoires sur la Respiration
et al Transpiration des Animaux” in “Les Maitres de la
Pensee Scienti~que.” Paris: Gauthier-Villaus et cie (eds.),
1920.
3. Smith JL. Pathological effects due to increase of oxygen
tension in air breathed. J Physiol 1899;24:19–35.
4. Gomberg M. An instance of trivalent carbon: Triphenylmethyl.
J Am Chem Soc 1900;22:757–761.
5. Gerschman R, Gilbert DL, Nye SW, Swyer P, Fenn WO.
Oxygen poisoning and X-irradiation: A mechanism in common.
Science 1954;119:623–626.
6. McCord JM, Fridovich I. Superoxide dismutase. An enzymatic
function for erythrocuprein (hemecuprein). J Biol
Chem 1969;244:6049–6055.
7. Halliwell B. Oxidants and human disease: Some new concepts.
FASEB J 1987;1:358–364.
8. Weiss SJ. Oxygen, ischemia and in_ammation. Acta Physiol
Scand 1986;548:9–31.
9. Singal PK, Kapur N, Dhillon KS, Beamish RE, Dhalla NS.
Role of free radicals in catecholamine-induced cardiomyopathy.
Can J Physiol Pharmacol 1982;60:1390–1397.
10. Singal PK, Deally CM, Weinberg LE. Subcellular effects of
adriamycin in the heart: A concise review. J Mol Cell
Cardiol 1987;19:817–828.
11. Bolli R. Oxygen-derived free radicals and postischemic myocardial
dysfunction (“stunned myocardium”). J Am Coll
Cardiol 1988;12:239–249.
12. Singal PK, Hill MF, Ganguly NK, Khaper N, Kirshenbaum
LA, Pichardo J. Role of oxidative stress in heart failure
subsequent to myocardial infarction. L’information Cardiologique.
1996;20:343–362.
13. Kaul N, Siveski-Iliskovic N, Hill M, Slezak J, Singal PK.
Free radicals and the heart. J Pharmacol Toxicol Meth
1993;30:55–67.
14. Singal PK, Petkau A, Gerrard JM, Hrushovetz S, Foerster
J. Free radicals in health and disease. Mol Cell Biochem
1988;84:121–122.
15. Singh N, Dhalla AK, Seneviratne CK, Singal PK. Oxidative
stress and heart failure. Mol Cell Biochem 1995;147:77–81.
16. Saran M, Michel C, Bors W. Reactions of NO with O2
2?.
Implications for the action of endothelium-derived relaxing
factor. Free Radical Res Commun 1989;10:221–226.
17. Singal PK, Kirshenbaum LA. A relative de~cit in antioxidant
reserve may contribute in cardiac failure. Can J
Cardiol 1990;6:47–49.
18. Singal PK, Beamish RE, Dhalla NS. Potential oxidative
pathways of catecholamines in the formation of lipid peroxides
and genesis of heart disease. Adv Exp Med Biol
1983;161:391–401.
19. Kaneko M, Singal PK, Dhalla NS. Alterations in heart sarcolemmal
Ca21 binding activities due to oxygen radicals.
Basic Res Cardiol 1990;85:45–54.
20. Reeves JP, Bailey CA, Hale CC. Redox modi~cation of so-
Oxidative Stress and Heart Failure 117
dium-calcium exchange activity in cardiac sarcolemmal vesicles.
J Biol Chem 1986;201:4948–4955.
21. Kramer JH,Mak IT,Weglicki WB. Differential sensitivity of
canine cardiac sarcolemmal and microsomal enzymes to inhibition
by free radical induced lipid peroxidation. Circ Res
1984;55:120–124.
22. Dixon IM, Kaneko M, Hata T, Panagia V, Dhalla NS. Alterations
in cardiac membrane Ca11 transport during oxidative
stress. Mol Cell Biochem 1990;99:125–133.
23. Ferrari R, Ceconi C, Curello S, Guarnieri C, Calderera CM,
Albertini A, Visioli O. Oxygen-mediated myocardial damage
during ischemia and reperfusion: Role of the cellular defenses
against oxygen toxicity. J Mol Cell Cardiol
1985;17:93–945.
24. Fridovich I. The biology of oxygen radicals. Science 1978;20
1:875–880.
25. Ferrari R, Agnoletti L, Comini L, Gata G, Bachetti T,
Cargnoni A, Ceconi C, Curello S, Visioli O. Oxidative stress
during myocardial ischemia and heart failure. Eur Heart J
1998;19:B2–B11.
26. Lawrence RA, Burk RE. Species, tissue and subcellular
distribution of non-selenium dependent glutathione peroxidase
activity. J Nutr 1978;108:211–215.
27. Freeman BA, Crapo JD. Biology of disease. Free radicals
and tissue injury. Lab Invest 1982;47:412–425.
28. Packer L. Vitamin E is nature’s master antioxidant.
Scienti~c American Science and Medicine 1994;54–63.
29. Packer L. Protective role of vitamin E in biological systems.
Am J Clin Nutr 1991;53:1050–1055.
30. Singal PK, Ghani RA-A; Khaper N, Palace V, Hill MF. Antioxidant
adaptations and cardiac dysfunction: Involvement of
vitamin E. J Adapt Med 1997;1:5–15.
31. Ozer NK, Boscoboinik A, Azzi A. New roles of low density
lipoproteins and vitamin E in the pathogenesis of
atherosclerosis. Biochem Mol Bio Int 1995;35:117–124.
32. Packer JE, Slater TF, Watson RL. Direct observation of a
free radical interaction between vitamin E and vitamin C.
Nature 1979;278:737–738.
33. Foote CS, Denny RW. Chemistry of singlet oxygen VII.
Quenching by b-carotene. J Am Chem Soc
1968;90:6233–6235.
34. Riemersma RA. Epidemiology and the role of antioxidants
in preventing coronary heart disease:A brief overview. Proc
Nutr Soc 1994;53:59–65.
35. Palace VP, Khaper N, Qin Q, Singal PK. Antioxidant potentials
of vitamin A and carotenoids and their relevance to
heart disease. Free Rad Biol Med 1999 26:746–761.
36. Clark IA, Cowden WB, Hunt NH. Free radical-induced pathology.
Medicinal Research Review 1985;5:297–332.
37. Reed DJ. Glutathione: Toxicological implications. Ann Rev
Pharmacol Toxicol 1990;30:603–631.
38. Reed DJ. Glutathione depletion and susceptibility. Pharmacol
Rev 1984;36:35S–33S.
39. Verma A, Hill M, Bhayana S, Pichardo J, Singal PK. Role of
glutathione in acute myocardial adaptation. In: Adaptation
Biology and Medicine: Subcellular Basis, Sharma BK,
Takeda N, Ganguly NK, Singal PK, eds. New Delhi, India:
Narosa Publishers, Vol. 1, 1997;399–408.
40. Ames BN, Cathcart R, Schivears E, Hochstein P. Uric acid
provides an antioxidant defense in humans against oxidant
and radical caused aging and cancer. Proc Natl Acad Sci
USA 1981;78:6858–6862.
41. Bernier M, Hearse DJ, Manning AS. Reperfusion-induced
arrhythmias and oxygen-derived free radicals: Studies with
“anti-free radical” interventions and a free radical-generating
system in the isolated perfused rat heart. Circ Res
1986;58:331–340.
42. Chopra K, Singh M, Kaul N, Andrabi KI, Ganguly NK.
Decrease of myocardial infarct size with desferrioxamine:
Possible role of oxygen free radicals in its ameliorative effect.
Mol Cell Biochem 1992;113:71–76.
43. Forsmark P, Aberg F, Norling B, Nordenbrand K, Dallner
G, Ernster L. Inhibition of lipid peroxidation by ubiquinol in
submitochondrial particles in the absence of vitamin E.
FEBS Lett 1991;285:39–43.
44. Halliwell B, Gutteridge JMC. Lipid peroxidation, oxygen
radicals, cell damage and antioxidant therapy. Lancet
1984;1396–1397.
45. Kappus H. Lipid peroxidation: Mechanisms, analysis, enzymology
and biological relevance. In: Oxidative Stress, Sies
H, ed. London: Academic Press, 1985:273–303.
46. Ceconi C, Cargnoni A, Pasini E, Condorelli E, Curello S,
Ferrari R. Evaluation of phospholipid peroxidation as
malondialdehyde during myocardial ischemia and reperfusion
injury. Am J Physiol 1991;260:H1057–H1061.
47. Sies H, ed. Oxidative Stress, Oxidants and Antioxidants.
London and New York: Academic Press, 1991.
48. Arroyo CM, Kramer JH, Dickens BF, Weglicki WB.
Identi~cation of free radicals in myocardial ischemia/reperfusion
by spin trapping with nitron DMPO. FEBS Lett
1987;221:101–104.
49. Garlick PB, Davies MJ, Hearse DJ, Slater TF. Direct detection
of free radicals in the reperfused rat heart using electron
spin resonance spectroscopy. Circ Res 1987;61:757–760.
50. Currello S, Ceconi C, de Giuli F, Panzali AF, Milanesi B,
Calarco M, Pardini A, Marzollo P, Al~eri O, Messineo F,
Ferrari R. Oxidative stress during reperfusion of human
hearts: Potential sources of oxygen free radicals. Cardiovasc
Res 1995;29:118–125.
51. Levy Y, Bartha P, Ben-Amotz A, Brook JG, Danker G, Lin
S, Hammerman H. Plasma antioxidants and lipid peroxidation
in acute myocardial infarction and thrombolysis. J Am
Coll Nutr 1998;17:373–341.
52. Jeroudi MO, Hartely CJ, Bolli R. Myocardial reperfusion
injury: Role of oxygen free radicals and potential therapy
with antioxidants. Am J Cardiol 1994;73:2B–7B.
53. Ferrari R, Al~eri O, Currello S, Ceconi C, Cargnoni A, Marzollo
P, Pardini A, Caradonna E, Visioli O. Occurrence of
oxidative stress during reperfusion of the human heart. Circulation
1990;81:201–211.
54. Palace V, Kumar D, Hill MF, Khaper N, Singal PK. Regional
differences in non-enzymatic antioxidants in the heart under
control and oxidative stress conditions. J Mol Cell Cardiol
1999 31:193–202.
55. Jolly SR, Kane WJ, Bailie MB, Abrams GD, Lucchesi BR.
Canine myocardial reperfusion injury: Its reduction by the
combined administration of superoxide dismutase and
catalase. Circ Res 1984;54:277–285.
56. Chen EP, Bittner HB, Davis RD, Van Trigt P, Folz RJ.
Physiologic effects of extracellular superoxide dismutase
transgene overexpression on myocardial function after ischemia
and reperfusion injury. J Thorac Cardiovasc Surg
1998;115:450–458.
57. Klein HH, Lindert PS, Buchwald A, Nerbendalh K, Kreuzer
H. Intracoronary superoxide dismutase for the treatment of
reperfusion injury. A blind randomized placebo-controlled
trial in ischemic-reperfused porcine hearts. Basic Res
Cardiol 1988;83:141–148.
118 Singal, Khaper, Palace, and Kumar
58. Shattock MJ. Do we know the mechanism of myocardial
stunning? Basic Res Cardiol 1998;93:145–149.
59. Bolli R. Causative role of oxyradicals in myocardial stunning:
A proven hypothesis. Basic Res Cardiol
1998;93:156–172.
60. Li Q, Bolli R, Qiu Y, Tang XL, Murphee SS, French BA.
Gene therapy with extracellular superoxide dismutase attenuates
myocardial stunning in conscious rabbits. Circulation
1998;98:1438–1448.
61. Francis GS, Goldsmith SR, Cohn JN. Relationship of exercise
capacity to resting left ventricular performance and
basal plasma norepinephrine levels in patients with congestive
heart failure. Am Heart J 1982;104:725–731.
62. Rathore N, John S, Kale M, Bhatnagar D. Lipid peroxidation
and antioxidant enzymes in isoproterenol-induced oxidative
stress in rat tissues. Pharmacol Res 1998;38:297–303.
63. Kirshenbaum LA, GuptaM, Thomas TP, Singal PK. Antioxidant
protection against adrenaline-induced arrhythmias in
rats with chronic heart hypertrophy. Can J Cardiol
1990;6:71–74.
64. Singal PK, Tong J. Vitamin E de~ciency accentuates
adriamycin-induced cardiomyopathy and cell surface
changes. Mol Cell Biochem 1988;84:163–171.
65. Doroshow JH. Effect of anthracycline antibiotics on oxygen
radical formation in rat heart. Cancer Res 1983;43:460–472.
66. Siveski-Iliskovic N, Kaul N, Singal PK. Probucol promotes
endogenous antioxidants and provides protection against
adriamycin-induced cardiomyopathy in rats. Circulation
1994;89:2829–2835.
67. Singal PK, Iliskovic N, Li T, Kumar D. Adriamycin
cardiomyopathy: Pathophysiology and prevention. FASEB
J 1997;11:931–936.
68. Singal PK, Iliskovic N. Doxorubicin-induced cardiomyopathy.
N Eng J Med 1998;339:900–905.
69. Myers CE, McGuire WP, Liss RH, In~rm I, Grutzinger K,
Young RC. Adriamycin: The role of lipid peroxidation in
cardiac toxicity and tumour response. Science 1977;19:
165–167.
70. Kaul N, Siveski-Iliskovic N, Thomas TP, Hill M, Singh N,
Singal PK. Probucol improves antioxidant activity and
modulates development of diabetic cardiomyopathy. Nutrition
1995;11:551–554.
71. Paolisso G, D’Amore A, Giugliano D, Ceriello A, Varricchio
M, D’Onofrio F. Pharmacological doses of vitamin E improve
insulin action in healthy subjects and non-insulin-dependent
diabetic patients. Am J Clin Nutr 1993;57:650–656.
72. Giugliano D, Ceriello A, Paolisso G. Diabetes mellitus, hypertension,
and cardiovascular disease: Which role for oxidative
stress? Metabolism 1995;44:363–368.
73. Wohaieb SA,GodinDV.Alterations in free radicals tissue-defense
mechanisms in streptozotocin-induced diabetes in rat.
Effects of insulin treatment. Diabetes 1987;36:1014–1018.
74. Gupta M, Singal PK. Higher antioxidative capacity during a
chronic stable heart hypertrophy. Circ Res 1989;64:398–406.
75. Kirshenbaum LA, Singal PK. Increase in endogenous antioxidant
enzymes protects hearts against reperfusion injury.
Am J Physiol 1993;2265:H484–H497.
76. Dhalla AK, Singal PK. Antioxidant changes in hypertrophied
and failing guinea pig hearts. Am J Physiol 1994;
266(Heart & Circ Physiol 36):H1280–H1285.
77. Dhalla AK, Hill MF, Singal PK. Role of oxidative stress in
transition of hypertrophy to heart failure. J Am Coll
Cardiol 1996;28:506–514.
78. Kirshenbaum LA, Hill M, Singal PK. Endogenous antioxidants
in isolated hypertrophied cardiac myocytes and hypoxia-
reoxygenation injury. J Mol Cell Cardiol 1995;27:
263–272.
79. Prasad K, Gupta JB, Kalra J, Lee P, Mantha SV, Bharadwaj
B. Oxidative stress as a mechanism of cardiac failure in
chronic volume overload in canine model. JMol Cell Cardiol
1996;28:375–385.
80. Hill MF, Singal PK. Antioxidant and oxidative stress
changes during heart failure subsequent to myocardial infarction
in rats. Am J Pathol 1996;148:291–300.
81. Palace VP, Hill MF, Farahmand F, Singal PK. Mobilization
of antioxidant vitamin pools and hemodynamic function following
myocardial infarction. Circulation 1999a;9:121–126.
82. Hill MF, Singal PK. Right and left myocardial antioxidant
responses during heart failure subsequent to myocardial
infarction. Circulation 1997;96:2414–2420.
83. Khaper N, Singal PK. Effects of afterload reducing drugs on
the pathogenesis of antioxidant changes and congestive
heart failure in rats. J Am Coll Cardiol 1997;29:856–861.
84. Khaper N, Hill MF, Pichardo J, Singal PK. Effects of captopril
on myocardial oxidative stress changes in post-MI rats.
In: Angiotensin II Blockade, Dhalla NS, Zahradka, P, Dixon
IMC, Beamish RE. Boston: Kluwer Academic Publishers,
1998; 527–536.
85. Axford-Gately RA, Wilson GJ. Reduction of experimental
myocardial infarct size by oral administration of alpha tocopherol.
Cardiovasc Res 1991;25:89–92.
86. Mickle DA, Li RK, Weisel RD. Myocardial salvage with
trolox and ascorbic acid for an acute evolving infarction.
Ann Thorac Surgery 1989;47:553–557.
87. Massey KD, Burton KP. Alpha-tocopherol attenuates myocardial
membrane-related alterations resulting from ischemia-
reperfusion. Am J Physiol 1989;256:H1192–1199.
88. Klein HH, Pich S, Lindert S, Nibendahl K, Niedman P,
Kreuzer H. Combined treatment with vitamins E and C in
experimental myocardial infarction in pigs. Am Heart J
1989;118:667–673.
89. Klein HH, Pich S, Lindert S, Nebendahl K, Niedman P.
Failure of chronic high dose oral vitamin E treatment to
protect the ischemic-reperfused porcine heart. J Mol Cell
Cardiol 1993;25:103–112.
90. Belch JJ, Bridges AB, Scott N, Chopra M. Oxygen free
radicals and congestive heart failure. Br Heart J
1991;65:245–248.
91. McMurray J, Mclay J, Chopra M, Bridges A, Belch JJF.
Evidence for enhanced free radical activity in chronic congestive
heart failure secondary to coronary artery disease.
Am J Cardiol 1990;65:1261–1262.
92. Diaz-Velez CR. Garcia-Castineiras S, Mendoza-Ramos E,
Hernandez-Lopez E, Increased malondialdehyde in peripheral
blood of patients with congestive heart failure. Am
Heart J 1996;131:146–152.
93. Sobotka PA, Brottman MD, Weitz Z, Birnbaum AJ, Skosey
JL, Zarling EJ. Elevated breath pentane in heart failure
reduced by free radical scavenger. Free Rad Biol Med
1993;14:643–647.
94. Weitz ZW, Birnbaum AJ, Sobotka S, Zarling EJ, Skosey JL.
High breath concentration during acute myocardial infarction.
Lancet 1991;337:933–935.
95. Charney RH, Levy DK, Kalman J, Buchholz E, et al. Free
radical activity increased with NYHA class in congestive
heart failure. J Am Coll Cardiol 1997;29:930–939.
96. Keith M, Geranmayegan A, Sole MJ, Kurian R, Robinson A,
Omran AS, Jeejeebhoy KN. Increased oxidative stress in
Oxidative Stress and Heart Failure 119
patients with congestive heart failure. J Am Coll Cardiol
1998;31:1352–1356.
97. Yucel D, Aydogdu S, Cehreli S, Saydam G, CanatanH, Senes
M, Cigdem Topkaya B, Nebioglu S. Increased oxidative
stress in dilated cardiomyopathic heart failure. Clin Chem
1998;44:148–154.
98. Rimm EB, Stampfer MJ, Ascherio A, Giovannucci E, Colditz
GA, Willet WC. Vitamin E consumption and the risk of
coronary disease in men. N Engl J Med 1993;328:1450–1456.
99. Stampfer MJ, Hennekens CH, Manson JE, Colditz GA, Rosner
B, Willett WC. Vitamin E consumption and the risk of
coronary disease in women. N Engl J Med 1993;328:
1444–1449.
100. Singh RB, Niaz MA, Rastogi S. Usefulness of antioxidant
vitamins in suspected acute myocardial infarction (the Indian
experiment of infarct survival -3). Am J Cardiol
1996;77:232–236.
101. Stephens NG, Parsons A, Scho~eld PM, Kelly F, Cheeseman
K, Mitchinson MJ. Randomised controlled trial of vitamin E
in patients with coronary disease: Cambridge Heart Antioxidant
Study (CHAOS). Lancet 1996;347:781–786.
102. Kok FJ, de Bruijn AM, Vermeeren R, Hofman A, van Laar
A, de Bruin M, Hermus RJ, Valkenburg HA. Serum selenium,
vitamin antioxidants and cardiovascular mortality: A
nine year follow-up study in the Netherlands. Am J Clin
Nutr 1987;45:462–468.
103. Hense HW, Stender M, Bors W, Keil V. Lack of an association
between serum vitamin E and myocardial infarction in
a population with high vitamin E levels. Atherosclerosis
1993;103:21–28.
104. Gottlieb RA. Burleson KO, Kloner RA, Babior BM, Engler
RL. Reperfusion injury induces apoptosis in rabbit
cardiomyocytes. J Clin Invest 1994;94:1621–1628.
105. Kajstura J, Liu Y, Baldini A, Li B, Olivetti G, Leri A, Anversa
P. Coronary artery constriction in rats: necrotic and
apoptotic myocyte death. Am J Cardiol 1998;82:30K–41K.
106. Teiger E, Than VD, Richard L, Wisnewsky C, Tea BS, Gaboury
L, Tremblay J, Schwartz K, Hamet P. Apoptosis in
pressure-overload induced heart hypertrophy in the rat. J
Clin Invest 1996;97:2891–2897.
107. Buttke TM, Sandstrom PA. Oxidative stress as a mediator
of apoptosis. Immunology Today 1994;15:7–10.
108. Saraste A, Pulkki K, Kallajoki M, Henriksen K, Parvinen M,
Voipo-Pulkki L-M. Apoptosis in human acute myocardial
infarction. Circulation 1997;95:320–323.
109. Narula J, Haider N, Virmani R, DiSalvo TG, Kolodgie FD,
Hajjar RJ, Schmidt U, Semigran MJ, Dec GW, Khaw BA.
Apoptosis in myocytes in end-stage heart failure. N Engl J
Med 1996;335:1182–1189.
110. Olivetti G, Abbi R, Quaini F, Kajstura J, ChengW, Nitahara
JA, Quaini E, DeLoreto C, Beltrami CA, Krajewski S, Reed
JC, Anversa P. Apoptosis in the failing human heart.N Engl
J Med 1997;336:1131–1141.
111. Lebovitz RM, Zhang H, Vogel H, Cartwright J Jr, Dionne L,
Lu N, Huang S, Matzuk MM. Neurodegeneration, myocardial
injury and perinatal death in mitochondrial superoxide
dismutase-de~cient mice. Proc Natl Acad Sci 1996;93:
9782–9787.
112. Kirshenbaum LA, de Moissac D. The bcl-2 gene product
prevents programmed cell death of ventricular myocytes.
Circulation 1997;96:1580–1585.
113. Hockenbery DM, Oltavi ZN, Yin XM, Milliman CL, Korsmeyer
ST. Bcl-2 functions in an antioxidant pathway to prevent
apoptosis. Cell 1993;75:241–251.
114. Krown KA, Page MT, Nguyen C, Zechner D, Gutierrez V,
Comstock KL, Glembotski CC, Quintana PJ, Sabbadini RA.
Tumor necrosis factor alpha-induced apoptosis in cardiac
myocytes. Involvement of the sphingolipid signaling cascade
in cardiac cell death. J Clin Invest 1996;98:2854–2865.
115. Bozkurt B, Kribbs SB, Chubb FJ Jr, Michael LH, Didenko
VV, Hornsby PJ, Seta Y, Oral H, Spinale FG, Mann DL.
Pathophysiologically relevant concentrations of tumor necrosis
factor-a promote progressive left ventricular dysfunction
and remodelling in rats. Circulation 1998;97:1382–1391.
116. Levine B, Kalman J, Mayer L, Fillit HM, Packer M. Elevated
circulating levels of tumor necrosis factor in congestive
heart failure. N Eng J Med 1990;323:236–241.
117. Givertz MM, Colucci WS.New targets for heart failure therapy:
endothelin, in_ammatory cytokines, and oxidative
stress. Lancet 1998;352(Suppl 1):34–38.
118. Blum A, Miller H. Role of cytokines in heart failure. Am
Heart J 1998;135:181–186.
119. Bryant D, Becker L, Richardson J, Shelton J, Franco F,
Peshock R, Thompson M, Giroir B. Cardiac failure in transgenic
mice with myocardial expression of tumor necrosis
factor-alpha. Circulation 1998;97:1375–1381.
120. Nakamura K, Fushimi K, Kouchi H, Mihara K, Miyazaki M,
Ohe T, Namba M. Inhibitory effects of antioxidants on neonatal
rat cardiac myocyte hypertrophy induced by tumor
necrosis factor-a and angiotensin II. Circulation
1998;98:794–799.
121. Meldrum DR, Dinarello CA, Cleveland JC Jr, Cain BS,
Shames BD, Meng X, Harken AH. Hydrogen peroxide induces
tumor necrosis factor a-mediated cardiac injury by a
p38 nitrogen-activated protein kinase-dependent mechanism.
Surgery 1998;124:291–296.
120 Singal, Khaper, Palace, and Kumar
Understanding and Prospective
OSxinidgaatli,v KehSatpreers,s PaanldacHe,eaanrtd FKauilmuraer
Pawan K. Singal, Neelam Khaper, Vince Palace,
and Dinender Kumar
Institute of Cardiovascular Sciences, St. Boniface General
Hospital Research Centre and Department of Physiology,
Faculty of Medicine, University of Manitoba, Winnipeg. Canada
Abstract. Our knowledge of the pathophysiology of heart
failure has advanced far beyond the classic concept of the
hemodynamic overload model and associated neurohumoral
changes. Current interest lies in understanding the fundamentals
of cellular defects to identify new molecular targets
for therapies. Some of the new target sites being explored
are in_ammatory cytokines, nitric oxide, oxidative
stress and apoptosis during the remodelling processes such
as cardiac hypertrophy and dilation. The present review
provides an overview of the chemistry/biochemistry of free
radicals as well as a discussion of some defense mechanisms
that have evolved and adapted to combat these toxic oxygen
species. The probable role of oxidative stress in the
pathogenesis of heart failure both from animal data and
heart failure patients is also presented.
Key Words. oxygen radicals, antioxidants, cardiac dysfunction
The Oxygen Story
Although we have witnessed a profound development
in the understanding of oxygen toxicity in heart disease
in the past three decades, the story of oxygen
toxicity started more than two centuries ago [1]. It was
Lavoisier, in 1785, who made the ~rst observation that
oxygen has two main effects, i.e. it supports life but it
also has toxic side effects [2]. More than a century later,
in 1899, Lorrain Smith demonstrated that increased
oxygen tension results in lung congestion in mice, rats
and pig [3]. At the beginning of the twentieth century,
Gomberg demonstrated the presence of triphenylmethyl
molecule as a radical species in organic chemistry
[4]. Subsequently, it was theorized that the damaging
effects of O2 were due to the formation of the
superoxide anion [5].
The pioneering work of McCord and Fridovich regarding
the discovery of superoxide dismutase [6], inspired
biologists and clinicians to study the role of free
radicals in biology and medicine. Thus, a large body
of evidence has accumulated showing that biological
systems are capable of producing a variety of reactive
oxidants which play an important role in various human
diseases [7,8]. Animal studies on catecholamineinduced
cardiomyopathy [9], adriamycin-induced
cardiomyopathy [10] and ischemia-reperfusion injury
[11] have now provided solid evidence for the role of
free radicals in the mediation of cardiac injury [1,12].
The present review provides some basic information
on free radicals and antioxidants and their signi~cance
in the pathogenesis of heart failure.
Oxygen Free Radicals
Free radicals are highly reactive atoms or molecules
with an unpaired electron in their outer orbits. The
production of free radicals occurs either by the addition
or by the removal of an electron in a reduction/
oxidation reaction. Since oxygen has two electrons
with parallel spin in its outermost shell, it is
characterized as a diradical which requires four electrons
to be completely reduced to water [13–15]. Oxygen
is also the terminal acceptor of electrons for oxidative
phosphorylation and this tetravalent reduction
is associated with the production of high energy phosphates
(Fig. 1). However, sequential univalent reduction
results in the formation of reactive oxygen intermediates
[7,8,13–15]. In the univalent reduction
pathway, the addition of a single electron to molecular
oxygen results in the production of superoxide anion
radical (O2
2?). The addition of another electron to the
O2
2? results in the formation of peroxide anion which
protonates to form hydrogen peroxide (H2O2). The latter
is not a radical by itself, but is capable of causing
cell damage by interacting with transition metals such
as iron. A single electron reduction of H2O2 results in
the formation of the hydroxyl radical (?OH) which is
highly reactive, has an extremely short half-life, and
therefore has a very limited diffusion capacity [13–15].
The addition of a fourth electron ~nally results in the
formation of water. The ~rst excited state of O2 is a
singlet oxygen (1O2) which can also initiate oxygen
Address for correspondence: Dr. Pawan K. Singal, Institute of
Cardiovascular Sciences, St. Boniface General Hospital Research
Centre, 351 Tache Avenue, R3022,Winnipeg, MB R2H 2A6, Canada.
Email: psingal@sbrc.umanitoba.ca
111
Heart Failure Reviews 1999;4:111–120
© Kluwer Academic Publishers. Boston. Printed in U.S.A.
radical chain reactions [13,14]. This univalent electron
reduction reaction is illustrated in ~gure 1.
Reactive oxygen intermediates such as O2
2?, H2O2,
?OH and 1O2 are called activated oxygen species and are
collectively known as partially reduced forms of oxygen
(PRFO) [13]. Superoxide radical reacts with nitric
oxide during reperfusion to form peroxynitrite which
also has a harmful effect by opposing the vasodilatory
effect of nitric oxide [16]. These reactive species can
interact with macromolecules and initiate free radical
chain reactions resulting in membrane and cell damage
[13–15,17].
Free Radical Mediated Cell Injury
The targets of free radical attack are the cell membranes
and subcellular organelles. The lipid peroxidation
chain reaction is initiated by the removal of a
hydrogen atom from the unsaturated site in a fatty
acid resulting in the production of a lipid radical. This
radical can further react with other neighbouring
polyunsaturated fatty acids (PUFA) to propagate the
reaction [13,18]. The addition of an oxygen molecule
to these lipid radicals results in the formation of lipid
peroxides. Free radical-induced lipid peroxidation has
been suggested to alter membrane structure and function
[13–15]. Considerable evidence also suggests that
PRFO can modify protein structure and function ultimately
affecting cell metabolism. In this regard, proteins
rich in sulphydryl groups are highly susceptible
to free radical attack [13]. In the myocardium, oxygen
radicals have been shown to effect Na1/Ca11 exchange,
Na1-K1 ATPase and Ca11 ATPase activities
[19–22]. Free radicals can also attack the nucleic acids
by producing base damage, single strand breaks, adducts
and chromosomal aberrations. Such modi~cations
have been shown to cause cellular abnormalities
such as mutations and cell death [12–15].
Fig. 1. A diagramatic representation of the production of oxygen free radicals by univalent reduction pathway. Different antioxidants
(enzymatic and non-enzymatic) in the biological system may offer protection against free radical-induced injury.
112 Singal, Khaper, Palace, and Kumar
The Antioxidant Defense System in
the Heart
Under normal physiological conditions, the tissue concentration
of free radicals is maintained by a system of
enzymatic and non-enzymatic antioxidants that have
evolved and been conserved during the evolution of
aerobic life and constitute “antioxidant reserve” in the
heart [17].
Enzymatic Antioxidants
Superoxide dismutase (SOD)
This enzyme is the ~rst line of defense against free radical
attack. There are several different types ofSODthat
differ based on the metallic prosthetic group attached
within the protein structure. The most common forms
are CuZnSOD (molecular weight 32,000) which is present
in the cytoplasm and MnSOD (molecular weight
80,000) found in the mitochondria. Superoxide dismutase
activity has been reported to be signi~cantly less in
the heart than in the liver [23–25]. It was the discovery
of SOD which led to the realization that O2
2? is formed in
vivo in living organisms [6]. Two superoxide anions can
react together to produce a spontaneous dismutation,
however, this reaction is much faster in the presence of
SOD (23109M21 sec21) [13,23–25].
Glutathione peroxidase
This selenium-dependent enzyme (molecular weight
~84,000), which is present mainly in the cytoplasm, represents
the second line of defense against free radicalmediated
damage. It catalyzes the reduction of hydrogen
peroxides using glutathione (GSH) as a source of
reducing power and forming oxidized glutathione
(GSSG), water and/or alcohol. This enzyme is present in
relatively high concentrations in the human heart
[13,23,25] and is therefore considered an important antioxidant
enzyme in that organ. A non selenium-dependent
form of GSHPx, which is non-speci~c to H2O2, but
which dismutates organic peroxides is also present [26].
Catalase
This enzyme (molecular weight 240,000) is also very
important in the metabolism of hydrogen peroxide produced
by SOD. Present at relatively low concentrations
in the heart, it converts H2O2 to water and oxygen.
However, the difference between catalase and
glutathione peroxidase is that GSHPx is more effective
at low concentrations of H2O2, i.e., in lM range,
whereas catalase is more effective at the mM concentrations
of H2O2 [13,15,27].
Non-enzymatic antioxidants
Non-enzymatic antioxidants include vitamins such as
tocopherols, ascorbate and carotenes as well as other
biological molecules including glutathione, uric acid
and metal binding proteins.
Vitamin E
Vitamin E functions as one of the major antioxidant
defense systems in our body. Vitamin E is a generic
term that includes a group of eight structurally related
compounds. These are further sub-divided into two
groups: tocopherols and trienols. Of these, d-a-tocopherol
is the most common type of vitamin E absorbed
from the human diet [28]. It is a strong biological antioxidant.
Because of its lipophillicity, vitamin E offers
maximum protection against free radical attack in cellular
and subcellular membranes. it reacts with free
radicals, yielding lipid hydroperoxides which can be
removed by the GSHPx enzyme system. In this reaction,
vitamin E donates a hydrogen atom, resulting in
the generation of a hydroperoxide and vitamin E radical.
This effectively terminates lipid peroxide-mediated
chain reaction and is therefore called a “chain
breaking antioxidant” [1,13,28–30]. Vitamin E also
functions synergistically with ascorbic acid to terminate
free radical chain reactions. Recent studies have
reported a new role for vitamin E that is independent
of its antioxidant property. These include inhibition of
smooth muscle cell (SMC) proliferation and growth
during atherosclerosis, occurring mainly as a result of
its ability to activate the release of TGF-b [31].
Vitamin C
Vitamin C is a water-soluble molecule which quenches
reactive oxygen metabolites directly, with the resultant
formation of dehydroascorbate. Vitamin C is also
thought to play an important role in the regeneration
of a tocopherol. It is present in micromolar concentrations
in the cytoplasm as well as in serum [13,32].
b carotene
b carotene, a precursor of vitamin A, is known to
quench active oxygen species. These reactions operate
maximally at low-oxygen tension as compared to vitamin
E which reacts at high oxygen tension [33].
Epidemiological studies have shown that increased
levels of b carotene and other carotenoids are associated
with a decreased risk of cardiovascular diseases
[34,35].
Glutathione
Glutathione is a tripeptide which is present in high
concentrations in the majority of the eukaryotic cells.
One of its important functions is to protect cells
against peroxides generated during aerobic metabolism.
It undergoes redox cycling between the reduced
(GSH) and oxidized (GSSG) forms [13,36,37]. In the
heart, glutathione is predominantly (.95%) in the
GSH form. It also acts as a cosubstrate for GSHPx
and plays an important role in defending against free
radical-mediated lipid peroxidation. This results in the
Oxidative Stress and Heart Failure 113
increased formation of GSSG. Depletion of GSH to
20–30% of its normal concentration renders the cell
susceptible to free radical attack [38,39]. The redox
ratio, which is the ratio of reduced to oxidized glutathione
(GSH/GSSG) is used as a sensitive index of
oxidative stress. Speci~cally, an increase in the redox
ratio indicates reduced oxidative stress, and vice versa
[1,13–15].
Other Biological Molecules
Uric acid, a waste product of purine metabolism, has
been shown to directly interact with ?OH and prevent
oxidation [40], suggesting that there is antioxidant potential
for this molecule in biological systems [13]. Iron
chelators, such as desferrioxamine, inhibit lipid peroxidation
and reduces the injury associated with ischemia-
reperfusion [41,42]. Ubiquinone also acts as a
potent inhibitor of lipid peroxidation by directly
quenching free radicals [43]. Various metal binding
proteins such as ferritin, transferrin, metallothioneins
and ceruloplasmin are also known to inhibit lipid peroxidation
[13,44].
Methods of Measuring Free
Radical-Mediated Damage
Free radical-mediated damage includes oxidative
modi~cation of cellular proteins, lipids and nucleic acids.
Lipid peroxidation is a free radical-mediated process,
which involves the formation of lipid peroxides
within cell membranes and organelles [13,44,45]. Lipid
peroxidation is detected through the estimation of
malondialdehyde (MDA) either by UV light, HPLC or
the thiobarbituric acid (TBA) method [13,45,46]. Direct
detection of free radicals is done by ESR spectroscopy,
although with this technique it is not possible to detect
low concentrations of free radicals [13]. Other methods
of assessing oxidative stress include determination of
diene conjugates, measurement of gases such as ethane,
pentane and isoprotanes in exhaled air, measuring
the redox ratio and the content and activity of endogenous
antioxidants and quantifying lipid hydroperoxides.
Although the MDA assay has been shown to lack
speci~city, it may detect saturated and unsaturated
aldehydes as well as other non lipid-related substances
[46], when used in conjunction with studies of the redox
ratio as well as antioxidant activity, it provides meaningful
information [1,12].
Oxidative Stress and Antioxidants in
Heart Failure
The classic de~nition of oxidative stress includes “a disturbance
in the prooxidant and antioxidant balance, in
favour of the former” [47]. The most common pathological
conditions that are attributed to oxidative stress
mediated damage are atherosclerosis, diabetes, cancer,
arrhythmia, rheumatoid arthritis, in_ammatory bowel
disease, and several neurodegenerative diseases. In
fact, this list of pathological conditions related to oxidative
stress continues to grow. In recent years, substantial
evidence has accumulated from acute and chronic
studies to suggest the role of antioxidants and oxidative
stress in the pathogenesis of heart dysfunction and
failure both in animal studies and from clinical trials
[12,13,25]. Early studies on catecholamine-induced
cardiomyopathy [9,18], adriamycin-cardiomyopathy
[10] and ischemia-reperfusion injury [11] provided the
basis for further investigation on understanding free
radical involvement in cardiac injury [1,12,13].
Acute Studies
Ischemia-reperfusion (I/R) is one of the most extensively
studied models of acute heart failure. Increased
free radical production and depressed antioxidant reserve
has been documented to have serious functional
consequences after I/R. Many studies have reported
the production of free radicals in reperfused hearts in
animals [25,48,49] and humans [50,51]. Studies on isolated
perfused rat hearts showed increased production
of H2O2 during ischemia as well as early reperfusion
[52]. An inverse correlation between oxidative stress
and cardiac function has also been documented in patients
who were subjected to global ischemia and
reperfusion during coronary artery bypass graft surgery
[53]. In isolated perfused rabbit hearts, a decline
in developed force during ischemia was accompanied
by a progressive decline in tissue glutathione content
and in the redox ratio indicating increased oxidative
stress [23]. A decrease in non-enzymatic antioxidants
such as tocopherol, retinol and ascorbic acid and redox
ratio and an increase in lipid peroxides has also recently
been reported in rat hearts subjected to 45 min
of ischemia and 15 min of reperfusion [54]. A reduction
in infarct size by SOD and catalase administration was
also reported in dogs that underwent 90 min of ischemia
and 24 hrs of reperfusion [55]. Similarly, another
study using transgenic mice, which overexpressed
MnSOD, demonstrated improved cardiac
function and decreased release of lactate dehydrogenase
in mice hearts subjected to ischemia-reperfusion
[56]. Lack of protection with antioxidants has also
been reported in some studies. SOD was found ineffective
in modulating ischemia reperfusion-induced
changes in pig hearts [57].
There is now overwhelming evidence that oxidative
stress also plays a major role in myocardial stunning
[11]. It has recently been suggested that approximately
80–85% of stunning can be attributed to free
radicals and the rest (15–20%) is due to Ca11 overload
[58]. Antioxidant therapy suppressed the production of
free radicals and attenuated myocardial stunning, suggesting
a cause and effect relationship [11,59]. An ele-
114 Singal, Khaper, Palace, and Kumar
gant study by Li and colleagues, using adenovirus-mediated
gene transfer technique to introduce SOD in the
rabbits, demonstrated protection against myocardial
stunning [60].
Chronic Heart Failure
Catecholamine-induced cardiomyopathy
Increased plasma levels and decreased tissue levels of
catecholamines have been reported in acute MI and
heart failure conditions [61]. Excess catecholamines
have been shown to cause arrhythmias as well as
cardiomyopathy [9]. Production of free radicals upon
autooxidation of catecholamines play a critical role in
cardiomyopathy [9,18]. A recent study reported increased
lipid peroxidation in isoproterenol administered
rat hearts [62]. Pretreatment of rats with vitamin
E reduced catecholamine-induced arrhythmia and
other functional changes [63]. Conversely, animals fed
a vitamin E de~cient diet were found to be more susceptible
to adriamycin- [64] and catecholamine-induced
cardiomyopathic changes [18].
Adriamycin Cardiomyopathy
It is now well established that adriamycin
cardiomyopathy is associated with increased free radicals
and decreased antioxidant status in the heart
[65–68]. Direct evidence for free radical involvement
was provided by studies which showed that vitamin
E-treated mice were more resistant to adriamycin
cardiotoxicity [69]. Moreover, rats maintained on a vitamin
E de~cient diet were more susceptible to
adriamycin cardiotoxicity [10,64]. Treatment with
probucol, an antioxidant as well as a lipid-lowering
drug, modulated the pathogenesis of heart failure due
to adriamycin [66,67].
Diabetic Cardiomyopathy
Evidence is available to suggest the role of increased
oxidative stress and depressed antioxidant enzyme activities
in the pathogenesis of diabetic cardiomyopathy.
A decrease in myocardial SOD and catalase activity
and an increase in oxidative stress has been demonstrated
in STZ-induced diabetic rats [70]. Furthermore,
probucol treatment in these rats resulted in improved
cardiac function [70]. Similarly, some other
studies reported reduced oxidative stress upon vitamin
E supplementation in animals and patients [71–73].
Hypertrophy and Heart Failure
An increase in endogenous antioxidant enzyme activities
and a decrease in lipid peroxidation have been
reported in the hypertrophy stage induced by chronic
pressure overload in rats [74,75] and guinea pigs
[76,77]. Myocytes isolated from hypertrophied rat
hearts also showed increased antioxidants and reduced
lipid peroxidation [78]. The heart failure stage in
guinea pigs was characterized by depressed cardiac
function, dyspnea, as well as lung and liver congestion.
At this stage, a signi~cant decline in myocardial SOD
and GSHPx activities and a decrease in the redox ratio
were indicative of increased oxidative stress [76]. Antioxidant
treatment of the guinea pigs with vitamin E
decreased oxidative stress and delayed the occurrence
of heart failure [77]. In a chronic volume overload
model in dogs, decrease in contractility was associated
with an increase in MDA and a decrease in antioxidant
enzyme activity. Vitamin E treatment modulated some
of these changes [79].
Myocardial Infarction and
Congestive Heart Failure
Recent data from our laboratory have demonstrated
the role of oxidative stress in the pathogenesis of heart
failure following myocardial infarction (MI) [1,12].
Changes in myocardial antioxidants as well as oxidative
stress have been described in the surviving myocardium
of rats subjected to MI. These changes correlated
with cardiac function at different stages of failure
[80]. In this study, maintenance of hemodynamic function
in early stages (non-failure stage) was accompanied
by a signi~cant decrease in oxidative stress and
lipid peroxidation while the antioxidant reserve was
maintained. In late stages, where hemodynamic function
was depressed, the myocardial antioxidants
GSHPx, catalase, SOD and vitamin E were also
signi~cantly decreased while oxidative stress was increased
[80]. In addition to the myocardium, the levels
of vitamin E and A were also depleted in the storage
organs liver and kidney in rats with severe heart failure
[81]. Supplementation of these rats with vitamin E
before subjecting them to coronary artery ligation surgery
modulated these changes [81].
In a study of regional changes in the two ventricles
during the sequelae of heart failure, the antioxidant
de~cit and an increase in oxidative stress occurred ~rst
in the left ventricle [80]. In moderate to severe heart
failure stages, these changes also occurred in the right
ventricle [82]. Thus, oxidative stress and antioxidant
changes correlated with depressed cardiac function in
the respective ventricles. In another study, using the
same animal model, we reported that improved cardiac
function after treatment with the afterload reducing
drugs captopril or prazosin was related to the maintenance
of myocardial endogenous antioxidant status and
decrease in oxidative stress [83,84].
Antioxidant vitamins such as vitamin C, carotenoids
and vitamin E have been shown to decrease lipid peroxidation
and reduce atherogenesis and the risk of
coronary heart disease. Pretreatment with vitamin E
limited myocardial necrosis [85–87] while combined
Oxidative Stress and Heart Failure 115
pretreatment with vitamins E and C in ischemia-reperfusion
settings was found to protect the myocardium
and reduce the infarct size in pigs [88]. On the other
hand, pigs supplemented with oral vitamin E did not
show reduction in infarct size after ischemia-reperfusion
[89].
Clinical Studies
An increase in oxidative stress has recently also been
documented in heart failure patients. Malondialdehyde
levels were found to be signi~cantly higher in congestive
heart failure patients [90–92]. Increased breath
pentane (a byproduct of lipid peroxidation) levels were
reported in exhaled air of CHF patients and treatment
with captopril attenuated this rise and improved the
patient’s clinical condition [93,94]. Furthermore, it has
also been demonstrated that the increase in lipid peroxidation
correlates with the severity of heart failure
[92,95,96]. In addition to an increase in free radical
activity, a decrease in enzymatic and non-enzymatic
antioxidants has also been reported in patients with
congestive heart failure [90–92,96]. In another recent
study, Yucel and colleagues reported a signi~cant decrease
in blood glutathione and erythrocyte SOD activity
and an increase in lipid peroxidation in dilated
cardiomyopathic heart failure patients [97]. All of these
clinical studies provided strong support for the involvement
of oxidative stress in the pathogenesis of
heart failure.
Various clinical trials have examined the bene~cial effects
of antioxidant vitamins in MI and heart failure
conditions. The Health Professional Follow-up Study
[98] and the Nurses Health Study [99] found a decrease
in the incidence of coronary artery disease in men and
women supplemented with vitamin E. In another
study, a cocktail containing antioxidant vitamins A, C,
E and b-carotene resulted in a decrease in oxidative
stress as well as the infarct size in MI patients [100].
The Cambridge Heart Antioxidant Study (CHAOS)
reported a substantial decrease in the incidence of MI
in patients receiving vitamin E [101]. On the other
hand, some studies found no correlation between serum
alpha-tocopherol concentration and subsequent
episodes of MI on cardiovascular disease related death
[102,103].
Oxidative Stress, Apoptosis and
Heart Failure
Although the molecular mechanisms involved in initiating
oxidative stress are not known, some recent
studies have suggested the role of transcription factors
such as NFjB and cytokines in linking oxidative stress
to remodelling processes, such as apoptosis (Fig. 2).
Findings from several in vitro studies and animal models
suggest that apoptosis occurs in response to ischemia-
reperfusion, myocardial infarction, and chronic
pressure overload [104–107], all of which are conditions
that generate oxidative stress [12,13]. More recently,
the loss of myocytes through apoptosis or programmed
cell death, has been reported in the infarct regions of
myocardium from MI patients [108] as well as in patients
with end-stage heart failure [109,110]. The importance
of oxidative stress in apoptosis is also
con~rmed by the observation that knock-out mice lacking
MnSOD die early due to cardiomyopathy as compared
to normal mice [111]. Mechanistic investigations
of apoptosis suggest that tumor suppression protein
p53 triggers and Bcl2 inhibits the process in cardiomyocytes
[107,112]. The mechanism of action of Bcl2 for the
prevention of apoptosis has also been suggested to be
mediated by an antioxidant pathway whereby Bcl2
gene product interacts with MnSOD and inhibits apoptosis
[113]. Cytokines as potent inducers of apoptosis
have also recently been suggested in CHF conditions
[114]. Although the role of oxidative stress in apoptosis
and heart failure patients have been documented, the
exact contribution of apoptosis in heart failure still
remains to be established. Some studies suggest that
Fig. 2. Events linking oxidative stress and heart failure in
cardiac stress conditions.
116 Singal, Khaper, Palace, and Kumar
apoptosis contributes to only 0.2% of the total
cardiomyocyte death at any given time [110]. Furthermore,
the underlying molecular mechanisms that regulate
this remodelling event are still not fully understood.
However, more details are provided in other
papers in this focussed issue.
Cytokines and Oxidative Stress in
Heart Failure
Increasing evidence from both animal [115] and human
studies (116) have reported elevated levels of cytokines
such as tumor necrosis factor a (TNF-a), interleukin-
1a (IL-1a) and atrial naturietic factor in MI
and failure conditions (Fig. 2). Increased concentrations
of these cytokines were shown to have a direct
relation to the severity of failure [25,116,117]. Recent
studies suggest that the deleterious effect of TNF-a
is receptor-mediated and it also directly activates nitric
oxide, which is cytotoxic to myocardial cells by
virtue of its ability to produce free radicals and cause
apoptosis [118] (Fig. 2). The pathological potential of
TNF-a is demonstrated in a study where transgenic
mice overexpressing TNF-a in the heart developed
cardiomyopathy associated with apoptosis [119]. In a
recent study, it was demonstrated that TNF-a and
Ang II induce hypertrophy in cultured neonatal cardiac
myocytes by virtue of producing free radicals and
antioxidants such as BHA, vitamin E and catalase inhibited
this effect of TNF-a and Ang II by scavenging
the radicals [120]. Another new concept suggests that
TNF-a not only produces PRFO, but is also activated
by hydrogen peroxide, in the presence of a P38MAP
kinase [121]. Further studies are required to clarify
this point. A detailed discussion on these aspects is
presented in other articles in this focussed issue of
the journal.
Conclusions
Although there is convincing evidence from both animal
studies and patients that oxidative stress plays a
very important role in the pathogenesis of heart failure
there are not enough clinical trials which have assessed
the use of antioxidant therapy in the treatment of
heart failure. The laboratory ~ndings described here
suggest newer target sites for the prevention of heart
failure as well as better management of patients by
using appropriate antioxidant therapies.
Acknowledgments
Research reported here was done with grant support from the
Medical Research Council of Canada and the Heart and Stroke
Foundation of Manitoba. Dr. Singal is supported by a career
award from the Medical Research Council of Canada, Dr. Palace
by a fellowship from the Manitoba Health Research Council and
Ms. Khaper by the Heart and Stroke Foundation of Canada.
References
1. Singal PK, Khaper N, Palace V, Kumar D. The role of oxidative
stress in the genesis of heart disease. Cardiovasc Res
1998;40:426–432.
2. Lavoisier A-L. Alterations qu’eprouve 1’air resire. Recueil
des memoires de Lavoisier. 1785, Read to the Societe de
Medicine. Reprinted as part of “Memoires sur la Respiration
et al Transpiration des Animaux” in “Les Maitres de la
Pensee Scienti~que.” Paris: Gauthier-Villaus et cie (eds.),
1920.
3. Smith JL. Pathological effects due to increase of oxygen
tension in air breathed. J Physiol 1899;24:19–35.
4. Gomberg M. An instance of trivalent carbon: Triphenylmethyl.
J Am Chem Soc 1900;22:757–761.
5. Gerschman R, Gilbert DL, Nye SW, Swyer P, Fenn WO.
Oxygen poisoning and X-irradiation: A mechanism in common.
Science 1954;119:623–626.
6. McCord JM, Fridovich I. Superoxide dismutase. An enzymatic
function for erythrocuprein (hemecuprein). J Biol
Chem 1969;244:6049–6055.
7. Halliwell B. Oxidants and human disease: Some new concepts.
FASEB J 1987;1:358–364.
8. Weiss SJ. Oxygen, ischemia and in_ammation. Acta Physiol
Scand 1986;548:9–31.
9. Singal PK, Kapur N, Dhillon KS, Beamish RE, Dhalla NS.
Role of free radicals in catecholamine-induced cardiomyopathy.
Can J Physiol Pharmacol 1982;60:1390–1397.
10. Singal PK, Deally CM, Weinberg LE. Subcellular effects of
adriamycin in the heart: A concise review. J Mol Cell
Cardiol 1987;19:817–828.
11. Bolli R. Oxygen-derived free radicals and postischemic myocardial
dysfunction (“stunned myocardium”). J Am Coll
Cardiol 1988;12:239–249.
12. Singal PK, Hill MF, Ganguly NK, Khaper N, Kirshenbaum
LA, Pichardo J. Role of oxidative stress in heart failure
subsequent to myocardial infarction. L’information Cardiologique.
1996;20:343–362.
13. Kaul N, Siveski-Iliskovic N, Hill M, Slezak J, Singal PK.
Free radicals and the heart. J Pharmacol Toxicol Meth
1993;30:55–67.
14. Singal PK, Petkau A, Gerrard JM, Hrushovetz S, Foerster
J. Free radicals in health and disease. Mol Cell Biochem
1988;84:121–122.
15. Singh N, Dhalla AK, Seneviratne CK, Singal PK. Oxidative
stress and heart failure. Mol Cell Biochem 1995;147:77–81.
16. Saran M, Michel C, Bors W. Reactions of NO with O2
2?.
Implications for the action of endothelium-derived relaxing
factor. Free Radical Res Commun 1989;10:221–226.
17. Singal PK, Kirshenbaum LA. A relative de~cit in antioxidant
reserve may contribute in cardiac failure. Can J
Cardiol 1990;6:47–49.
18. Singal PK, Beamish RE, Dhalla NS. Potential oxidative
pathways of catecholamines in the formation of lipid peroxides
and genesis of heart disease. Adv Exp Med Biol
1983;161:391–401.
19. Kaneko M, Singal PK, Dhalla NS. Alterations in heart sarcolemmal
Ca21 binding activities due to oxygen radicals.
Basic Res Cardiol 1990;85:45–54.
20. Reeves JP, Bailey CA, Hale CC. Redox modi~cation of so-
Oxidative Stress and Heart Failure 117
dium-calcium exchange activity in cardiac sarcolemmal vesicles.
J Biol Chem 1986;201:4948–4955.
21. Kramer JH,Mak IT,Weglicki WB. Differential sensitivity of
canine cardiac sarcolemmal and microsomal enzymes to inhibition
by free radical induced lipid peroxidation. Circ Res
1984;55:120–124.
22. Dixon IM, Kaneko M, Hata T, Panagia V, Dhalla NS. Alterations
in cardiac membrane Ca11 transport during oxidative
stress. Mol Cell Biochem 1990;99:125–133.
23. Ferrari R, Ceconi C, Curello S, Guarnieri C, Calderera CM,
Albertini A, Visioli O. Oxygen-mediated myocardial damage
during ischemia and reperfusion: Role of the cellular defenses
against oxygen toxicity. J Mol Cell Cardiol
1985;17:93–945.
24. Fridovich I. The biology of oxygen radicals. Science 1978;20
1:875–880.
25. Ferrari R, Agnoletti L, Comini L, Gata G, Bachetti T,
Cargnoni A, Ceconi C, Curello S, Visioli O. Oxidative stress
during myocardial ischemia and heart failure. Eur Heart J
1998;19:B2–B11.
26. Lawrence RA, Burk RE. Species, tissue and subcellular
distribution of non-selenium dependent glutathione peroxidase
activity. J Nutr 1978;108:211–215.
27. Freeman BA, Crapo JD. Biology of disease. Free radicals
and tissue injury. Lab Invest 1982;47:412–425.
28. Packer L. Vitamin E is nature’s master antioxidant.
Scienti~c American Science and Medicine 1994;54–63.
29. Packer L. Protective role of vitamin E in biological systems.
Am J Clin Nutr 1991;53:1050–1055.
30. Singal PK, Ghani RA-A; Khaper N, Palace V, Hill MF. Antioxidant
adaptations and cardiac dysfunction: Involvement of
vitamin E. J Adapt Med 1997;1:5–15.
31. Ozer NK, Boscoboinik A, Azzi A. New roles of low density
lipoproteins and vitamin E in the pathogenesis of
atherosclerosis. Biochem Mol Bio Int 1995;35:117–124.
32. Packer JE, Slater TF, Watson RL. Direct observation of a
free radical interaction between vitamin E and vitamin C.
Nature 1979;278:737–738.
33. Foote CS, Denny RW. Chemistry of singlet oxygen VII.
Quenching by b-carotene. J Am Chem Soc
1968;90:6233–6235.
34. Riemersma RA. Epidemiology and the role of antioxidants
in preventing coronary heart disease:A brief overview. Proc
Nutr Soc 1994;53:59–65.
35. Palace VP, Khaper N, Qin Q, Singal PK. Antioxidant potentials
of vitamin A and carotenoids and their relevance to
heart disease. Free Rad Biol Med 1999 26:746–761.
36. Clark IA, Cowden WB, Hunt NH. Free radical-induced pathology.
Medicinal Research Review 1985;5:297–332.
37. Reed DJ. Glutathione: Toxicological implications. Ann Rev
Pharmacol Toxicol 1990;30:603–631.
38. Reed DJ. Glutathione depletion and susceptibility. Pharmacol
Rev 1984;36:35S–33S.
39. Verma A, Hill M, Bhayana S, Pichardo J, Singal PK. Role of
glutathione in acute myocardial adaptation. In: Adaptation
Biology and Medicine: Subcellular Basis, Sharma BK,
Takeda N, Ganguly NK, Singal PK, eds. New Delhi, India:
Narosa Publishers, Vol. 1, 1997;399–408.
40. Ames BN, Cathcart R, Schivears E, Hochstein P. Uric acid
provides an antioxidant defense in humans against oxidant
and radical caused aging and cancer. Proc Natl Acad Sci
USA 1981;78:6858–6862.
41. Bernier M, Hearse DJ, Manning AS. Reperfusion-induced
arrhythmias and oxygen-derived free radicals: Studies with
“anti-free radical” interventions and a free radical-generating
system in the isolated perfused rat heart. Circ Res
1986;58:331–340.
42. Chopra K, Singh M, Kaul N, Andrabi KI, Ganguly NK.
Decrease of myocardial infarct size with desferrioxamine:
Possible role of oxygen free radicals in its ameliorative effect.
Mol Cell Biochem 1992;113:71–76.
43. Forsmark P, Aberg F, Norling B, Nordenbrand K, Dallner
G, Ernster L. Inhibition of lipid peroxidation by ubiquinol in
submitochondrial particles in the absence of vitamin E.
FEBS Lett 1991;285:39–43.
44. Halliwell B, Gutteridge JMC. Lipid peroxidation, oxygen
radicals, cell damage and antioxidant therapy. Lancet
1984;1396–1397.
45. Kappus H. Lipid peroxidation: Mechanisms, analysis, enzymology
and biological relevance. In: Oxidative Stress, Sies
H, ed. London: Academic Press, 1985:273–303.
46. Ceconi C, Cargnoni A, Pasini E, Condorelli E, Curello S,
Ferrari R. Evaluation of phospholipid peroxidation as
malondialdehyde during myocardial ischemia and reperfusion
injury. Am J Physiol 1991;260:H1057–H1061.
47. Sies H, ed. Oxidative Stress, Oxidants and Antioxidants.
London and New York: Academic Press, 1991.
48. Arroyo CM, Kramer JH, Dickens BF, Weglicki WB.
Identi~cation of free radicals in myocardial ischemia/reperfusion
by spin trapping with nitron DMPO. FEBS Lett
1987;221:101–104.
49. Garlick PB, Davies MJ, Hearse DJ, Slater TF. Direct detection
of free radicals in the reperfused rat heart using electron
spin resonance spectroscopy. Circ Res 1987;61:757–760.
50. Currello S, Ceconi C, de Giuli F, Panzali AF, Milanesi B,
Calarco M, Pardini A, Marzollo P, Al~eri O, Messineo F,
Ferrari R. Oxidative stress during reperfusion of human
hearts: Potential sources of oxygen free radicals. Cardiovasc
Res 1995;29:118–125.
51. Levy Y, Bartha P, Ben-Amotz A, Brook JG, Danker G, Lin
S, Hammerman H. Plasma antioxidants and lipid peroxidation
in acute myocardial infarction and thrombolysis. J Am
Coll Nutr 1998;17:373–341.
52. Jeroudi MO, Hartely CJ, Bolli R. Myocardial reperfusion
injury: Role of oxygen free radicals and potential therapy
with antioxidants. Am J Cardiol 1994;73:2B–7B.
53. Ferrari R, Al~eri O, Currello S, Ceconi C, Cargnoni A, Marzollo
P, Pardini A, Caradonna E, Visioli O. Occurrence of
oxidative stress during reperfusion of the human heart. Circulation
1990;81:201–211.
54. Palace V, Kumar D, Hill MF, Khaper N, Singal PK. Regional
differences in non-enzymatic antioxidants in the heart under
control and oxidative stress conditions. J Mol Cell Cardiol
1999 31:193–202.
55. Jolly SR, Kane WJ, Bailie MB, Abrams GD, Lucchesi BR.
Canine myocardial reperfusion injury: Its reduction by the
combined administration of superoxide dismutase and
catalase. Circ Res 1984;54:277–285.
56. Chen EP, Bittner HB, Davis RD, Van Trigt P, Folz RJ.
Physiologic effects of extracellular superoxide dismutase
transgene overexpression on myocardial function after ischemia
and reperfusion injury. J Thorac Cardiovasc Surg
1998;115:450–458.
57. Klein HH, Lindert PS, Buchwald A, Nerbendalh K, Kreuzer
H. Intracoronary superoxide dismutase for the treatment of
reperfusion injury. A blind randomized placebo-controlled
trial in ischemic-reperfused porcine hearts. Basic Res
Cardiol 1988;83:141–148.
118 Singal, Khaper, Palace, and Kumar
58. Shattock MJ. Do we know the mechanism of myocardial
stunning? Basic Res Cardiol 1998;93:145–149.
59. Bolli R. Causative role of oxyradicals in myocardial stunning:
A proven hypothesis. Basic Res Cardiol
1998;93:156–172.
60. Li Q, Bolli R, Qiu Y, Tang XL, Murphee SS, French BA.
Gene therapy with extracellular superoxide dismutase attenuates
myocardial stunning in conscious rabbits. Circulation
1998;98:1438–1448.
61. Francis GS, Goldsmith SR, Cohn JN. Relationship of exercise
capacity to resting left ventricular performance and
basal plasma norepinephrine levels in patients with congestive
heart failure. Am Heart J 1982;104:725–731.
62. Rathore N, John S, Kale M, Bhatnagar D. Lipid peroxidation
and antioxidant enzymes in isoproterenol-induced oxidative
stress in rat tissues. Pharmacol Res 1998;38:297–303.
63. Kirshenbaum LA, GuptaM, Thomas TP, Singal PK. Antioxidant
protection against adrenaline-induced arrhythmias in
rats with chronic heart hypertrophy. Can J Cardiol
1990;6:71–74.
64. Singal PK, Tong J. Vitamin E de~ciency accentuates
adriamycin-induced cardiomyopathy and cell surface
changes. Mol Cell Biochem 1988;84:163–171.
65. Doroshow JH. Effect of anthracycline antibiotics on oxygen
radical formation in rat heart. Cancer Res 1983;43:460–472.
66. Siveski-Iliskovic N, Kaul N, Singal PK. Probucol promotes
endogenous antioxidants and provides protection against
adriamycin-induced cardiomyopathy in rats. Circulation
1994;89:2829–2835.
67. Singal PK, Iliskovic N, Li T, Kumar D. Adriamycin
cardiomyopathy: Pathophysiology and prevention. FASEB
J 1997;11:931–936.
68. Singal PK, Iliskovic N. Doxorubicin-induced cardiomyopathy.
N Eng J Med 1998;339:900–905.
69. Myers CE, McGuire WP, Liss RH, In~rm I, Grutzinger K,
Young RC. Adriamycin: The role of lipid peroxidation in
cardiac toxicity and tumour response. Science 1977;19:
165–167.
70. Kaul N, Siveski-Iliskovic N, Thomas TP, Hill M, Singh N,
Singal PK. Probucol improves antioxidant activity and
modulates development of diabetic cardiomyopathy. Nutrition
1995;11:551–554.
71. Paolisso G, D’Amore A, Giugliano D, Ceriello A, Varricchio
M, D’Onofrio F. Pharmacological doses of vitamin E improve
insulin action in healthy subjects and non-insulin-dependent
diabetic patients. Am J Clin Nutr 1993;57:650–656.
72. Giugliano D, Ceriello A, Paolisso G. Diabetes mellitus, hypertension,
and cardiovascular disease: Which role for oxidative
stress? Metabolism 1995;44:363–368.
73. Wohaieb SA,GodinDV.Alterations in free radicals tissue-defense
mechanisms in streptozotocin-induced diabetes in rat.
Effects of insulin treatment. Diabetes 1987;36:1014–1018.
74. Gupta M, Singal PK. Higher antioxidative capacity during a
chronic stable heart hypertrophy. Circ Res 1989;64:398–406.
75. Kirshenbaum LA, Singal PK. Increase in endogenous antioxidant
enzymes protects hearts against reperfusion injury.
Am J Physiol 1993;2265:H484–H497.
76. Dhalla AK, Singal PK. Antioxidant changes in hypertrophied
and failing guinea pig hearts. Am J Physiol 1994;
266(Heart & Circ Physiol 36):H1280–H1285.
77. Dhalla AK, Hill MF, Singal PK. Role of oxidative stress in
transition of hypertrophy to heart failure. J Am Coll
Cardiol 1996;28:506–514.
78. Kirshenbaum LA, Hill M, Singal PK. Endogenous antioxidants
in isolated hypertrophied cardiac myocytes and hypoxia-
reoxygenation injury. J Mol Cell Cardiol 1995;27:
263–272.
79. Prasad K, Gupta JB, Kalra J, Lee P, Mantha SV, Bharadwaj
B. Oxidative stress as a mechanism of cardiac failure in
chronic volume overload in canine model. JMol Cell Cardiol
1996;28:375–385.
80. Hill MF, Singal PK. Antioxidant and oxidative stress
changes during heart failure subsequent to myocardial infarction
in rats. Am J Pathol 1996;148:291–300.
81. Palace VP, Hill MF, Farahmand F, Singal PK. Mobilization
of antioxidant vitamin pools and hemodynamic function following
myocardial infarction. Circulation 1999a;9:121–126.
82. Hill MF, Singal PK. Right and left myocardial antioxidant
responses during heart failure subsequent to myocardial
infarction. Circulation 1997;96:2414–2420.
83. Khaper N, Singal PK. Effects of afterload reducing drugs on
the pathogenesis of antioxidant changes and congestive
heart failure in rats. J Am Coll Cardiol 1997;29:856–861.
84. Khaper N, Hill MF, Pichardo J, Singal PK. Effects of captopril
on myocardial oxidative stress changes in post-MI rats.
In: Angiotensin II Blockade, Dhalla NS, Zahradka, P, Dixon
IMC, Beamish RE. Boston: Kluwer Academic Publishers,
1998; 527–536.
85. Axford-Gately RA, Wilson GJ. Reduction of experimental
myocardial infarct size by oral administration of alpha tocopherol.
Cardiovasc Res 1991;25:89–92.
86. Mickle DA, Li RK, Weisel RD. Myocardial salvage with
trolox and ascorbic acid for an acute evolving infarction.
Ann Thorac Surgery 1989;47:553–557.
87. Massey KD, Burton KP. Alpha-tocopherol attenuates myocardial
membrane-related alterations resulting from ischemia-
reperfusion. Am J Physiol 1989;256:H1192–1199.
88. Klein HH, Pich S, Lindert S, Nibendahl K, Niedman P,
Kreuzer H. Combined treatment with vitamins E and C in
experimental myocardial infarction in pigs. Am Heart J
1989;118:667–673.
89. Klein HH, Pich S, Lindert S, Nebendahl K, Niedman P.
Failure of chronic high dose oral vitamin E treatment to
protect the ischemic-reperfused porcine heart. J Mol Cell
Cardiol 1993;25:103–112.
90. Belch JJ, Bridges AB, Scott N, Chopra M. Oxygen free
radicals and congestive heart failure. Br Heart J
1991;65:245–248.
91. McMurray J, Mclay J, Chopra M, Bridges A, Belch JJF.
Evidence for enhanced free radical activity in chronic congestive
heart failure secondary to coronary artery disease.
Am J Cardiol 1990;65:1261–1262.
92. Diaz-Velez CR. Garcia-Castineiras S, Mendoza-Ramos E,
Hernandez-Lopez E, Increased malondialdehyde in peripheral
blood of patients with congestive heart failure. Am
Heart J 1996;131:146–152.
93. Sobotka PA, Brottman MD, Weitz Z, Birnbaum AJ, Skosey
JL, Zarling EJ. Elevated breath pentane in heart failure
reduced by free radical scavenger. Free Rad Biol Med
1993;14:643–647.
94. Weitz ZW, Birnbaum AJ, Sobotka S, Zarling EJ, Skosey JL.
High breath concentration during acute myocardial infarction.
Lancet 1991;337:933–935.
95. Charney RH, Levy DK, Kalman J, Buchholz E, et al. Free
radical activity increased with NYHA class in congestive
heart failure. J Am Coll Cardiol 1997;29:930–939.
96. Keith M, Geranmayegan A, Sole MJ, Kurian R, Robinson A,
Omran AS, Jeejeebhoy KN. Increased oxidative stress in
Oxidative Stress and Heart Failure 119
patients with congestive heart failure. J Am Coll Cardiol
1998;31:1352–1356.
97. Yucel D, Aydogdu S, Cehreli S, Saydam G, CanatanH, Senes
M, Cigdem Topkaya B, Nebioglu S. Increased oxidative
stress in dilated cardiomyopathic heart failure. Clin Chem
1998;44:148–154.
98. Rimm EB, Stampfer MJ, Ascherio A, Giovannucci E, Colditz
GA, Willet WC. Vitamin E consumption and the risk of
coronary disease in men. N Engl J Med 1993;328:1450–1456.
99. Stampfer MJ, Hennekens CH, Manson JE, Colditz GA, Rosner
B, Willett WC. Vitamin E consumption and the risk of
coronary disease in women. N Engl J Med 1993;328:
1444–1449.
100. Singh RB, Niaz MA, Rastogi S. Usefulness of antioxidant
vitamins in suspected acute myocardial infarction (the Indian
experiment of infarct survival -3). Am J Cardiol
1996;77:232–236.
101. Stephens NG, Parsons A, Scho~eld PM, Kelly F, Cheeseman
K, Mitchinson MJ. Randomised controlled trial of vitamin E
in patients with coronary disease: Cambridge Heart Antioxidant
Study (CHAOS). Lancet 1996;347:781–786.
102. Kok FJ, de Bruijn AM, Vermeeren R, Hofman A, van Laar
A, de Bruin M, Hermus RJ, Valkenburg HA. Serum selenium,
vitamin antioxidants and cardiovascular mortality: A
nine year follow-up study in the Netherlands. Am J Clin
Nutr 1987;45:462–468.
103. Hense HW, Stender M, Bors W, Keil V. Lack of an association
between serum vitamin E and myocardial infarction in
a population with high vitamin E levels. Atherosclerosis
1993;103:21–28.
104. Gottlieb RA. Burleson KO, Kloner RA, Babior BM, Engler
RL. Reperfusion injury induces apoptosis in rabbit
cardiomyocytes. J Clin Invest 1994;94:1621–1628.
105. Kajstura J, Liu Y, Baldini A, Li B, Olivetti G, Leri A, Anversa
P. Coronary artery constriction in rats: necrotic and
apoptotic myocyte death. Am J Cardiol 1998;82:30K–41K.
106. Teiger E, Than VD, Richard L, Wisnewsky C, Tea BS, Gaboury
L, Tremblay J, Schwartz K, Hamet P. Apoptosis in
pressure-overload induced heart hypertrophy in the rat. J
Clin Invest 1996;97:2891–2897.
107. Buttke TM, Sandstrom PA. Oxidative stress as a mediator
of apoptosis. Immunology Today 1994;15:7–10.
108. Saraste A, Pulkki K, Kallajoki M, Henriksen K, Parvinen M,
Voipo-Pulkki L-M. Apoptosis in human acute myocardial
infarction. Circulation 1997;95:320–323.
109. Narula J, Haider N, Virmani R, DiSalvo TG, Kolodgie FD,
Hajjar RJ, Schmidt U, Semigran MJ, Dec GW, Khaw BA.
Apoptosis in myocytes in end-stage heart failure. N Engl J
Med 1996;335:1182–1189.
110. Olivetti G, Abbi R, Quaini F, Kajstura J, ChengW, Nitahara
JA, Quaini E, DeLoreto C, Beltrami CA, Krajewski S, Reed
JC, Anversa P. Apoptosis in the failing human heart.N Engl
J Med 1997;336:1131–1141.
111. Lebovitz RM, Zhang H, Vogel H, Cartwright J Jr, Dionne L,
Lu N, Huang S, Matzuk MM. Neurodegeneration, myocardial
injury and perinatal death in mitochondrial superoxide
dismutase-de~cient mice. Proc Natl Acad Sci 1996;93:
9782–9787.
112. Kirshenbaum LA, de Moissac D. The bcl-2 gene product
prevents programmed cell death of ventricular myocytes.
Circulation 1997;96:1580–1585.
113. Hockenbery DM, Oltavi ZN, Yin XM, Milliman CL, Korsmeyer
ST. Bcl-2 functions in an antioxidant pathway to prevent
apoptosis. Cell 1993;75:241–251.
114. Krown KA, Page MT, Nguyen C, Zechner D, Gutierrez V,
Comstock KL, Glembotski CC, Quintana PJ, Sabbadini RA.
Tumor necrosis factor alpha-induced apoptosis in cardiac
myocytes. Involvement of the sphingolipid signaling cascade
in cardiac cell death. J Clin Invest 1996;98:2854–2865.
115. Bozkurt B, Kribbs SB, Chubb FJ Jr, Michael LH, Didenko
VV, Hornsby PJ, Seta Y, Oral H, Spinale FG, Mann DL.
Pathophysiologically relevant concentrations of tumor necrosis
factor-a promote progressive left ventricular dysfunction
and remodelling in rats. Circulation 1998;97:1382–1391.
116. Levine B, Kalman J, Mayer L, Fillit HM, Packer M. Elevated
circulating levels of tumor necrosis factor in congestive
heart failure. N Eng J Med 1990;323:236–241.
117. Givertz MM, Colucci WS.New targets for heart failure therapy:
endothelin, in_ammatory cytokines, and oxidative
stress. Lancet 1998;352(Suppl 1):34–38.
118. Blum A, Miller H. Role of cytokines in heart failure. Am
Heart J 1998;135:181–186.
119. Bryant D, Becker L, Richardson J, Shelton J, Franco F,
Peshock R, Thompson M, Giroir B. Cardiac failure in transgenic
mice with myocardial expression of tumor necrosis
factor-alpha. Circulation 1998;97:1375–1381.
120. Nakamura K, Fushimi K, Kouchi H, Mihara K, Miyazaki M,
Ohe T, Namba M. Inhibitory effects of antioxidants on neonatal
rat cardiac myocyte hypertrophy induced by tumor
necrosis factor-a and angiotensin II. Circulation
1998;98:794–799.
121. Meldrum DR, Dinarello CA, Cleveland JC Jr, Cain BS,
Shames BD, Meng X, Harken AH. Hydrogen peroxide induces
tumor necrosis factor a-mediated cardiac injury by a
p38 nitrogen-activated protein kinase-dependent mechanism.
Surgery 1998;124:291–296.
120 Singal, Khaper, Palace, and Kumar
lunedì 26 marzo 2012
venerdì 23 marzo 2012
Ca mammario precoce, Pet/Ct meglio di esami standard
Ca mammario precoce, Pet/Ct meglio di esami standard
L'esame Pet/Ct risulta più accurato dell'imaging convenzionale nella stadiazione del cancro mammario precoce a causa di una maggiore rilevazione di metastasi e tumori sincroni. Inoltre la stessa tecnica determina una modificazione del trattamento in un sostanziale numero di pazienti. È il verdetto di uno studio retrospettivo effettuato in Germania da Carolin Rieggerdell'università di Düsseldorf e collaboratori dell'università di Duisburg-Essen su 106 donne (età media: 57 anni) sottoposte a Fdg Pet/Ct whole-body e a imaging convenzionale (mammografia , Rm mammaria, radiografia del torace, scintigrafia ossea ed ecografie mammaria, ascellare ed epatica). Si è quindi messa a confronto l'accuratezza diagnostica della tecnica medico-nucleare con quella dell'algoritmo convenzionale; l'accuratezza è stata valutata in termini di tasso di detezione di tumore primario, corretta valutazione della focalità della lesione primaria, stadiazione T e tasso di rilevazione dei linfonodi e delle metastasi a distanza. Come standard di riferimento si è ricorso all'istopatologia e ai dati clinici o di imaging al follow-up. La Fdg Pet/Ct si è dimostrata significativamente più accurata nella detezione dei linfonodi ascellari e delle metastasi a distanza. Non si sono riscontrate differenze significative per altri parametri. Tumori sincroni o linfonodi extra-ascellari locoregionali o metastasi a distanza sono stati rilevati in 14 pazienti (13%) soltanto mediante la tecnica Fdg Pet/Ct. Sulla base dei reperti di quest'ultima metodica, inoltre, è stata modificata la gestione clinica di 15 donne (14%), di cui 3 avevano metastasi ai linfonodi ascellari, 5 a quelli extra-ascellari, 4 mostravano metastasi a distanza e 3 presentavano cancri sincroni.
Eur J Nucl Med Mol Imaging, 2012 Mar 6
L'esame Pet/Ct risulta più accurato dell'imaging convenzionale nella stadiazione del cancro mammario precoce a causa di una maggiore rilevazione di metastasi e tumori sincroni. Inoltre la stessa tecnica determina una modificazione del trattamento in un sostanziale numero di pazienti. È il verdetto di uno studio retrospettivo effettuato in Germania da Carolin Rieggerdell'università di Düsseldorf e collaboratori dell'università di Duisburg-Essen su 106 donne (età media: 57 anni) sottoposte a Fdg Pet/Ct whole-body e a imaging convenzionale (mammografia , Rm mammaria, radiografia del torace, scintigrafia ossea ed ecografie mammaria, ascellare ed epatica). Si è quindi messa a confronto l'accuratezza diagnostica della tecnica medico-nucleare con quella dell'algoritmo convenzionale; l'accuratezza è stata valutata in termini di tasso di detezione di tumore primario, corretta valutazione della focalità della lesione primaria, stadiazione T e tasso di rilevazione dei linfonodi e delle metastasi a distanza. Come standard di riferimento si è ricorso all'istopatologia e ai dati clinici o di imaging al follow-up. La Fdg Pet/Ct si è dimostrata significativamente più accurata nella detezione dei linfonodi ascellari e delle metastasi a distanza. Non si sono riscontrate differenze significative per altri parametri. Tumori sincroni o linfonodi extra-ascellari locoregionali o metastasi a distanza sono stati rilevati in 14 pazienti (13%) soltanto mediante la tecnica Fdg Pet/Ct. Sulla base dei reperti di quest'ultima metodica, inoltre, è stata modificata la gestione clinica di 15 donne (14%), di cui 3 avevano metastasi ai linfonodi ascellari, 5 a quelli extra-ascellari, 4 mostravano metastasi a distanza e 3 presentavano cancri sincroni.
Eur J Nucl Med Mol Imaging, 2012 Mar 6
giovedì 22 marzo 2012
Third Heart Sound
Physiology of the Third Heart Sound: Novel Insights
from Tissue Doppler Imaging
Sanjiv J. Shah, MD, Gregory M. Marcus, MD, Ivor L. Gerber, MD,
Barry H. McKeown, MD, Joshua C. Vessey, MD, Mark V. Jordan, MD,
Michele Huddleston, RN, MS, Elyse Foster, MD, Kanu Chatterjee, MB, and
Andrew D. Michaels, MD, MAS, San Francisco, California; and Salt Lake City, Utah
Background: The third heart sound (S3) is thought to be caused by the abrupt deceleration of left ventricular
(LV) inflow during early diastole, increased LV filling pressures, and decreased LV compliance. We sought to
determine whether the ratio of early mitral inflow velocity to diastolic velocity of the mitral annulus (E/E’) could
confirm the proposed mechanism of the S3.
Methods: A total of 90 subjects underwent phonocardiography, echocardiography, tissue Doppler imaging,
and left-sided heart catheterization.
Results: Phonocardiography detected an S3 in 21 patients (23%). Subjects with an S3 had lower ejection
fraction (P .0006) and increased E deceleration rate (P .0001), E/E’ (P .0001) and filling pressures
(P .0001). The phonocardiographic S3 confidence score correlated with E/E’ (r 0.46; P .0001) and E
deceleration rate (r 0.43, P .0001). Of the echocardiographic variables, only E/E’ was independently
associated with the S3 confidence score (P .009), independently of invasively determined LV filling
pressures (P .001).
Conclusions: The most important determinants of the pathologic S3 are an increased deceleration rate of
early mitral inflow, elevated LV filling pressures, and abnormal compliance of the myocardium as measured
by tissue Doppler imaging.
The third heart sound (S3) is an important auscultatory finding with
diagnostic and prognostic value in a variety of clinical conditions,
including symptomatic and asymptomatic left ventricular (LV) dysfunction,
1-5 valvular heart disease,6,7 acute myocardial infarction,8
and noncardiac surgery.9 The S3 occurs in early diastole, approximately
120 to 160 msec after the second heart sound, during the
rapid LV filling phase10 and deceleration of early mitral inflow.11,12
Although it has limited sensitivity, the S3 has high specificity for the
detection of LV dysfunction.13
Since its initial description by Potain,14-16 there has been speculation
regarding the underlying physiology of the S3.8,17 It was previously
believed that the S3 resulted from transient mitral valve closure
in early diastole18,19 or impact of the heart against the chest wall.20
Most now agree that an abrupt limitation of LV inflow during early
diastole causes vibration of the entire cardiohemic system resulting in
the S3 under both physiologic and pathologic conditions.8,10,11,17 An
increase in the volume and pressure of mitral inflow in early diastole,
coupled with an abnormally stiff, noncompliant ventricle, is the ideal
setting in which the pathologic S3 becomes audible.17 These hemodynamic
conditions increase the deceleration rate of early mitral
inflow, a finding that has also been associated with the S3.12,21,22
Echocardiographic measurement of the ratio of mitral inflow
velocity to diastolic velocity of the mitral annulus (E/E’) with tissue
Doppler imaging (TDI) may be an ideal method to confirm the
proposed mechanism of the S3. However, there have been no known
prior studies on TDI and the S3.17
We hypothesized that the pathologic S3 is associated with an
elevated E/E’ and that mitral inflow and mitral annulus velocity may
be important characteristics underlying the physiology of the S3. We
therefore undertook a comprehensive investigation of the physiology
of the pathologic S3 using computerized phonocardiography, B-type
natriuretic peptide (BNP) testing, Doppler echocardiography, and
invasive LV hemodynamics in adult patients undergoing cardiac
catheterization.
METHODS
Study Participants
We performed a cross-sectional study of 100 unselected adult patients
referred for nonurgent cardiac catheterization at the University
of California, San Francisco Medical Center. The research protocol
From the Division of Cardiology, Department of Medicine, University of California,
San Francisco, California; and Division of Cardiology, Department of Medicine,
University of Utah, Salt Lake City, Utah.
Potential conflicts of interest: None.
Dr. Michaels received an unrestricted educational grant from Inovise Medical, Inc.
(Portland, OR). Dr. Shah is supported by a Heart Failure Society of America
Research Fellowship Award.
Reprint requests: Andrew D. Michaels, MD, MAS, Division of Cardiology, University
of Utah, 30 North 1900 East, Room 4A100, Salt Lake City, UT 84132-2401
(E-mail: andrew.michaels@hsc.utah.edu).
0894-7317/$34.00
Copyright 2008 by the American Society of Echocardiography.
doi:10.1016/j.echo.2007.06.007
394
from Tissue Doppler Imaging
Sanjiv J. Shah, MD, Gregory M. Marcus, MD, Ivor L. Gerber, MD,
Barry H. McKeown, MD, Joshua C. Vessey, MD, Mark V. Jordan, MD,
Michele Huddleston, RN, MS, Elyse Foster, MD, Kanu Chatterjee, MB, and
Andrew D. Michaels, MD, MAS, San Francisco, California; and Salt Lake City, Utah
Background: The third heart sound (S3) is thought to be caused by the abrupt deceleration of left ventricular
(LV) inflow during early diastole, increased LV filling pressures, and decreased LV compliance. We sought to
determine whether the ratio of early mitral inflow velocity to diastolic velocity of the mitral annulus (E/E’) could
confirm the proposed mechanism of the S3.
Methods: A total of 90 subjects underwent phonocardiography, echocardiography, tissue Doppler imaging,
and left-sided heart catheterization.
Results: Phonocardiography detected an S3 in 21 patients (23%). Subjects with an S3 had lower ejection
fraction (P .0006) and increased E deceleration rate (P .0001), E/E’ (P .0001) and filling pressures
(P .0001). The phonocardiographic S3 confidence score correlated with E/E’ (r 0.46; P .0001) and E
deceleration rate (r 0.43, P .0001). Of the echocardiographic variables, only E/E’ was independently
associated with the S3 confidence score (P .009), independently of invasively determined LV filling
pressures (P .001).
Conclusions: The most important determinants of the pathologic S3 are an increased deceleration rate of
early mitral inflow, elevated LV filling pressures, and abnormal compliance of the myocardium as measured
by tissue Doppler imaging.
The third heart sound (S3) is an important auscultatory finding with
diagnostic and prognostic value in a variety of clinical conditions,
including symptomatic and asymptomatic left ventricular (LV) dysfunction,
1-5 valvular heart disease,6,7 acute myocardial infarction,8
and noncardiac surgery.9 The S3 occurs in early diastole, approximately
120 to 160 msec after the second heart sound, during the
rapid LV filling phase10 and deceleration of early mitral inflow.11,12
Although it has limited sensitivity, the S3 has high specificity for the
detection of LV dysfunction.13
Since its initial description by Potain,14-16 there has been speculation
regarding the underlying physiology of the S3.8,17 It was previously
believed that the S3 resulted from transient mitral valve closure
in early diastole18,19 or impact of the heart against the chest wall.20
Most now agree that an abrupt limitation of LV inflow during early
diastole causes vibration of the entire cardiohemic system resulting in
the S3 under both physiologic and pathologic conditions.8,10,11,17 An
increase in the volume and pressure of mitral inflow in early diastole,
coupled with an abnormally stiff, noncompliant ventricle, is the ideal
setting in which the pathologic S3 becomes audible.17 These hemodynamic
conditions increase the deceleration rate of early mitral
inflow, a finding that has also been associated with the S3.12,21,22
Echocardiographic measurement of the ratio of mitral inflow
velocity to diastolic velocity of the mitral annulus (E/E’) with tissue
Doppler imaging (TDI) may be an ideal method to confirm the
proposed mechanism of the S3. However, there have been no known
prior studies on TDI and the S3.17
We hypothesized that the pathologic S3 is associated with an
elevated E/E’ and that mitral inflow and mitral annulus velocity may
be important characteristics underlying the physiology of the S3. We
therefore undertook a comprehensive investigation of the physiology
of the pathologic S3 using computerized phonocardiography, B-type
natriuretic peptide (BNP) testing, Doppler echocardiography, and
invasive LV hemodynamics in adult patients undergoing cardiac
catheterization.
METHODS
Study Participants
We performed a cross-sectional study of 100 unselected adult patients
referred for nonurgent cardiac catheterization at the University
of California, San Francisco Medical Center. The research protocol
From the Division of Cardiology, Department of Medicine, University of California,
San Francisco, California; and Division of Cardiology, Department of Medicine,
University of Utah, Salt Lake City, Utah.
Potential conflicts of interest: None.
Dr. Michaels received an unrestricted educational grant from Inovise Medical, Inc.
(Portland, OR). Dr. Shah is supported by a Heart Failure Society of America
Research Fellowship Award.
Reprint requests: Andrew D. Michaels, MD, MAS, Division of Cardiology, University
of Utah, 30 North 1900 East, Room 4A100, Salt Lake City, UT 84132-2401
(E-mail: andrew.michaels@hsc.utah.edu).
0894-7317/$34.00
Copyright 2008 by the American Society of Echocardiography.
doi:10.1016/j.echo.2007.06.007
394
venerdì 16 marzo 2012
APPUNTI
Aumento di peso da beta-bloccanti nei pazienti scompensati
L'uso di beta-bloccanti e l'intensificazione del trattamento dello scompenso cardiaco si associano a un aumento di peso nell'insufficienza cronica congestizia. L'aumento, di solito colpisce, i soggetti non edematosi in classe funzionale Nyha (New York Heart Association) I e II. Il dato - rilevante considerando che esiste un'associazione inversa tra massa corporea e mortalità nei pazienti con scompenso cardiaco cronico - giunge da Kingston-upon-Hull (UK), dove Ben W. J. Boxall e Andrew L. Clarke, della University of Hull, hanno studiato 276 pazienti affetti da insufficienza cardiaca (età media: 71,3 anni; 72,8% maschi). Per prima cosa i partecipanti sono stati pesati. Alla presentazione nessuno di questi assumeva beta-bloccanti, ma tutti avevano iniziato ad assumerli entro il follow-up a 4 mesi. I soggetti sono stati pesati nuovamente dopo 1 anno. Vi è stato un incremento di peso e di indice di massa corporea. I pazienti in classe funzionale Nyha III o IV non hanno evidenziato modificazioni significative di peso, mentre quelli in classe I o II hanno fatto registrare un incremento di 1,62 kg. Nei pazienti senza edema periferico alla visita iniziale o a 1 anno, si registrava un maggiore incremento di peso.
J Card Fail, 2012; 18(3):233-7
Leohuck A et al. Ann Intern Med 2012; 156: 105-114Gold DR and Manson JE. Ann Intern Med 2012; 156: 156-157

Lakkireddy D et al. Feasibility and Safety of Dabigatran Versus Warfarin for Periprocedural Anticoagulation in Patients Undergoing Radiofrequency Ablation for Atrial Fibrillation Results From a Multicenter Prospective Registry.
J Am Coll Cardiol 2012; doi:10.1016/j.jacc.2011.12.014 (Published online 1 February 2012)

Heneghan C et al. The Lancet 2012; 379: 322-334

Hyeon-Cheol G et al. Six-Month Versus 12-Month Dual Antiplatelet Therapy After Implantation of Drug-Eluting Stents: The Efficacy of Xience/Promus Versus Cypher to Reduce Late Loss After Stenting (
Endocrinologia
Diabete negli ipertesi: meno danni cardiovascolari dai tiazidici
Nei soggetti ipertesi in terapia farmacologica, la comparsa di diabete correlato a un diuretico tiazidico determina minore impatto sugli eventi avversi cardiovascolari a lungo termine rispetto a quello determinato da altri antipertensivi (calcio-antagonisti e Ace-inibitori). È quanto emerso da un'estensione dello studio Allhat (Antihypertensive and Lipid-Lowering Treatment to Prevent Heart Attack Trial), coordinato da Joshua I. Barzilay del Kaiser Permanente of Georgia and Emory University School of Medicine, ad Atlanta (Usa). Sono stati selezionati 22.418 partecipanti allo studio principale, con diabete basale, diabete incidente (7,5% trattati con clortalidone, 5,6% con amlodipina, 4,3% con lisinopril), oppure senza diabete a un follow-up a 2 anni nel trial; tutti questi soggetti sono stati seguiti per un periodo medio di 6,9 anni tramite database nazionali. I partecipanti trattati con clortalidone affetti da diabete mellito - rispetto a quelli senza - hanno mostrato un rischio consistentemente minore, anche se non statisticamente significativo, di mortalità cardiovascolare, generale, non cardiovascolare e coronarica rispetto ai partecipanti in terapia con amlodipina o lisinopril con diabete incidente. In generale, i pazienti colpiti da diabete incidente hanno evidenziato un rischio coronarico superiore rispetto a quello dei soggetti senza diabete, rischio che in quelli trattati con clortalidone era significativamente inferiore rispetto a quelli in terapia con lisinopril.
Circ Cardiovasc Qual Outcomes, 2012 Mar 6.
Nefrologia
Più frequenti le apnee notturne, minore la funzione renale
Le apnee notturne sono frequenti nei pazienti con nefropatia cronica (Ckd), e aumentano con la riduzione della funzione renale. Quasi il 50% dei pazienti con Ckd e malattia renale allo stadio terminale (Esrd) sperimentano ipossia notturna, che può contribuire a perdita di funzionalità renale e ad aumento di rischio cardiovascolare. Il collegamento tra funzione respiratoria e renale è stato effettuato da un'équipe della university of Calgary (Canada) guidata da David D. M. Nicholl, che ha reclutato 254 pazienti da cliniche nefrologiche e unità di emodialisi per sottoporli a un monitoraggio notturno cardiopolmonare allo scopo di determinare la prevalenza di apnea notturna (indice di disturbo respiratorio =/>15) e ipossia notturna (saturazione d'ossigeno <90% per un monitoraggio =/>12%). I pazienti sono stati stratificati in 3 gruppi sulla base del tasso stimato di filtrazione glomerulare: eGfr =/>60 , Ckd (eGfr<60 non in dialisi) e Esrd (in emodialisi). La prevalenza di apnea notturna è risultata superiore nei pazienti con Ckd e Esrd.
Chest, 2012 Jan 5.EXCELLENT) Randomized, Multicenter Study. Circulation 2012; 125: 505-513
L'uso di beta-bloccanti e l'intensificazione del trattamento dello scompenso cardiaco si associano a un aumento di peso nell'insufficienza cronica congestizia. L'aumento, di solito colpisce, i soggetti non edematosi in classe funzionale Nyha (New York Heart Association) I e II. Il dato - rilevante considerando che esiste un'associazione inversa tra massa corporea e mortalità nei pazienti con scompenso cardiaco cronico - giunge da Kingston-upon-Hull (UK), dove Ben W. J. Boxall e Andrew L. Clarke, della University of Hull, hanno studiato 276 pazienti affetti da insufficienza cardiaca (età media: 71,3 anni; 72,8% maschi). Per prima cosa i partecipanti sono stati pesati. Alla presentazione nessuno di questi assumeva beta-bloccanti, ma tutti avevano iniziato ad assumerli entro il follow-up a 4 mesi. I soggetti sono stati pesati nuovamente dopo 1 anno. Vi è stato un incremento di peso e di indice di massa corporea. I pazienti in classe funzionale Nyha III o IV non hanno evidenziato modificazioni significative di peso, mentre quelli in classe I o II hanno fatto registrare un incremento di 1,62 kg. Nei pazienti senza edema periferico alla visita iniziale o a 1 anno, si registrava un maggiore incremento di peso.
J Card Fail, 2012; 18(3):233-7
Alte dosi di vitamina D per ridurre le esacerbazioni nel paziente con BPCO?
Bassi livelli sierici di 25-idrossivitamina D sono stati associati a più bassi livelli di FEV1, ad un alterato controllo immunologico ed a aumentato rischio di infiammazione delle vie aeree. Poiché molti pazienti con broncopneumopatia cronica ostruttiva (BPCO) presentano carenza di vitamina D, gli effetti della supplementazione di vitamina D potrebbe andare ben oltre la prevenzione dell'osteoporosi. L'obiettivo di un recente studio belga (randomizzato, monocentrico, in doppio cieco, controllato con placebo), è stato quello di valutare se la supplementazione con alte dosi di vitamina D potesse ridurre l'incidenza delle riacutizzazioni della BPCO. Sono stati arruolati nell'ospedale universitario di Leuven 182 pazienti con BPCO (da moderata a molto grave) e con una storia di recente esacerbazione, a cui sono stati somministrati 100.000 UI di vitamina D o di placebo ogni 4 settimane per 1 anno. L'esito primario era il tempo alla prima esacerbazione. Gli outcome secondari erano: tempo alla prima esacerbazione, tempo alla prima ospedalizzazione, tempo alla seconda esacerbazione, FEV1, qualità della vita e mortalità. Si è osservato un significativo aumento medio dei livelli di vitamina D nel gruppo trattati rispetto al gruppo placebo (media differenza tra i gruppi 30 ng/mL [95% CI, 27-33 ng/ml], p <0.001 ). Non vi è stata differenza significativa tra i due gruppi (hazard ratio 1.1 [CI 0.82-1.56], p = 0.41) nel tempo medio alla prima esacerbazione, tassi di riacutizzazione, FEV1, ospedalizzazione, qualità della vita e mortalità. Tuttavia un'analisi post hoc, effettuata in 30 partecipanti con grave deficit di vitamina D (livelli sierici di 25-[OH] D livelli inferiori a 10 ng/ml) al basale, ha mostrato una significativa riduzione delle esacerbazioni nel gruppo trattato con vitamina D (rate ratio 0.57 [CI 0.33-0.98], p = 0.042). La principale limitazione dello studio è costituita dall'essere stato condotto in un singolo centro con un piccolo campione di pazienti. Questo studio in cui il deficit severo di vitamina D è risultato essere un prerequisito per la risposta alla supplementazione nei pazienti BPCO ha sollecitato una discussione interessante tra gli editorialisti, in particolare sulla necessità di chiarire - anche con studi successivi - il rapporto tra rischi e benefici nella supplementazione con vit. D nei pazienti con disfunzione respiratoria.Leohuck A et al. Ann Intern Med 2012; 156: 105-114Gold DR and Manson JE. Ann Intern Med 2012; 156: 156-157

Attenzione all'uso del dabigatran nei pazienti con fibrillazione atriale candidati all'ablazione
Dopo l'alert degli inizi di gennaio (Dabigatran Association With Higher Disk of Acute Coronary Events. Meta-analysis of Noninferiority Randomized Controlled Trials. Ken Uchino et al. Arch Intern Med. Published online January 9, 2012. doi:10.1001/archinternmed.2011.1666), ecco un'altra segnalazione riguardante un potenziale svantaggio nell'utilizzo del dabigatran. Un gruppo di cardiologi americani ha segnalato sul numero di JACC del 24 gennaio che, in pazienti sottoposti ad ablazione di fibrillazione atriale (FA), l'uso periprocedurale di dabigatran aumenta significativamente il rischio di sanguinamento e/o di complicanze tromboemboliche rispetto all'utilizzo di warfarin. In questo studio prospettico multicentrico sono stati inclusi 290 pazienti con FA parossistica (età media 60 anni, 79% maschi), provenienti da 8 diversi centri degli USA, di cui 145 randomizzati ad assumere dabigatran ed altri 145 ad assumere warfarin nel periodo peri-procedurale. Come è possibile evincere dalle dueTabelle accluse- 3 complicanze tromboemboliche (2,1%) si sono verificate nel gruppo dabigatran e nessuna nel gruppo warfarin (p=0.25),
- il gruppo dabigatran ha presentato inoltre una incidenza significativamente più alta di sanguinamenti maggiori (6% vs 1%; p=0.019) e di sanguinamenti totali (14% vs 6%; p=0.009) rispetto al gruppo warfarin,
- inoltre, ad un'analisi di regressione multivariata, il dabigatran si è confermato un predittore indipendente di sanguinamento o complicanze tromboemboliche (odds ratio 2.76; 95% IC 1.22 a 6.25; p=0,01).
Lakkireddy D et al. Feasibility and Safety of Dabigatran Versus Warfarin for Periprocedural Anticoagulation in Patients Undergoing Radiofrequency Ablation for Atrial Fibrillation Results From a Multicenter Prospective Registry.
J Am Coll Cardiol 2012; doi:10.1016/j.jacc.2011.12.014 (Published online 1 February 2012)

L'auto-monitorizzazione della TAO
Gli antagonisti della vitamina K sono stati usati con successo per più di 50 anni nella terapia anticoagulante orale (TAO) dei pazienti a rischio di tromboembolismo venoso o arterioso, però la loro gestione non è semplice. Esistono a tale proposito gli ambulatori dedicati ma un notevole interesse ha destato l'introduzione di sistemi computerizzati che, previo addestramento, permettono al paziente stesso di gestire la sua coagulazione. Heneghan e collaboratori hanno fanno il punto su queste nuove metodiche con una metanalisi di 11 studi che avevano confrontato la TAO auto-monitorizzata con la TAO controllata in modo convenzionale. Ebbene, l'auto-monitorizzazione è risultata superiore alla "usual care", con una riduzione del rischio del 49% di eventi tromboembolici. I sanguinamenti sono stati simili in entrambi i gruppi e l'auto-monitorizzazione non ha avuto maggiori effetti sulla mortalità (ma neanche minori, in contrasto con altri report). Questi risultati sono migliori dell'auto-testing, in cui il test viene eseguito dal paziente ma la terapia viene regolata dal medico, però non si sono dimostrati uguali per tutti: infatti sono stati più evidenti nei soggetti di età inferiore a 55 anni e con protesi valvolari meccaniche, rispetto a quelli con fibrillazione atriale (FA) e più anziani, forse per la maggiore coscienza che questi pazienti hanno della loro malattia. Certamente bisogna considerare l'alternativa dei nuovi anticoagulanti (dabigratan, rivaroxiban, apixaban) che non necessitano di monitoraggio ed hanno risultati pari o superiori ai dicumarolici, ma ancora ci sono delle incertezze: per esempio vengono filtrati dal rene e quindi non vanno bene nell'insufficienza renale, non hanno antidoti e sono costosi. Inoltre non sono ancora chiari i loro outcomes nei pazienti con protesi meccaniche e FA. Come si vede non c'è, per ora, una soluzione unica: vanno valutati i singoli casi (accessibilità ai controlli, protesi meccaniche, età e affidabilità del paziente) e sarà il medico a decidere quale sarà la soluzione più razionale per il suo paziente.Heneghan C et al. The Lancet 2012; 379: 322-334

Duplice terapia antiaggregante dopo stent medicato: 6 o 12 mesi?
Alcuni colleghi cardiologi coreani hanno voluto verificare quale fosse il periodo di durata "ideale" della duplice terapia antiaggregante (DAPT) dopo il posizionamento di uno stent medicato: 6 o 12 mesi? Per farlo hanno valutato come endpoint primario il Target Vessel Failure (TVF), definito come endpoint composito di morte cardiaca, infarto miocardico o rivascolarizzazione del vaso ischemia-guidata a 12 mesi, in due gruppi di pazienti che avevano rispettivamente effettuato la duplice antiaggregazione per 6 o 12 mesi dopo l'impianto dello stent. La percentuale di TVF a 12 mesi è stata pari al- 4,8% nel gruppo in cui la DAPT è durata per 6 mesi
- 4,3% nel gruppo in cui la DAPT è stata prolungata per 12 mesi (dato indicativo di non inferiorità, visto che il limite superiore di 1 intervallo di confidenza bilaterale al 95% è risultato del 2.4%, p = 0.001 per la non-inferiorità, essendo il margine di non inferiorità predefinito del 4.0%).
Hyeon-Cheol G et al. Six-Month Versus 12-Month Dual Antiplatelet Therapy After Implantation of Drug-Eluting Stents: The Efficacy of Xience/Promus Versus Cypher to Reduce Late Loss After Stenting (
Maggior mortalità determinata dal consumo di carne rossa
Il consumo di carne rossa è associato a un maggiore rischio di mortalità totale o legata a malattia cardiovascolare o al cancro. La sua sostituzione nella dieta alimentare con altre sorgenti proteiche determina un minore rischio cardiovascolare. Ne è convinta An Pan che, a capo di un'èquipe di studiosi ad Harvard (Boston), ha coordinato l'analisi retrospettiva di dati provenienti da 2 coorti, una di 37.698 uomini, l'altra da 83.644 donne, tutti privi di malattie cardiovascolari od oncologiche al basale. Il regime dietetico è stato analizzato tramite questionari validati e aggiornati ogni 4 anni. Sono stati documentati 23.926 decessi (di cui 5.910 cardiovascolari e 9.464 per cancro) durante un follow-up di 2,96 milioni di anni-persona. Dopo aggiustamento multivariabile per i maggiori fattori di rischio comportamentali e dietetici, l'hazard ratio (Hr) della mortalità totale per l'aumento di una porzione al giorno di carne rossa non trasformata è stato di 1,13, e di 1,20 per quella trasformata. I valori corrispondenti per la mortalità cardiovascolare sono risultati 1,18 e 1,21, e quelli per la mortalità da cancro 1,10 e 1,16. Gli autori stimano che la sostituzione di 1 razione al giorno di altri cibi (pesce, pollame, nocciole, legumi, grano intero) al posto di una porzione al giorno di carne rossa si associ a una riduzione del rischio di mortalità dal 7% al 19%. Inoltre, si ritiene che, nel periodo osservato, il 9,3% delle morti tra gli uomini e il 7,6% dei decessi tra le donne possa essere prevenuto al termine se tutti gli individui avessero consumato meno di 0,5 razioni al giorno (circa 42 g/die) di carne rossa.
Arch Intern Med, 2012 Mar 12
Il consumo di carne rossa è associato a un maggiore rischio di mortalità totale o legata a malattia cardiovascolare o al cancro. La sua sostituzione nella dieta alimentare con altre sorgenti proteiche determina un minore rischio cardiovascolare. Ne è convinta An Pan che, a capo di un'èquipe di studiosi ad Harvard (Boston), ha coordinato l'analisi retrospettiva di dati provenienti da 2 coorti, una di 37.698 uomini, l'altra da 83.644 donne, tutti privi di malattie cardiovascolari od oncologiche al basale. Il regime dietetico è stato analizzato tramite questionari validati e aggiornati ogni 4 anni. Sono stati documentati 23.926 decessi (di cui 5.910 cardiovascolari e 9.464 per cancro) durante un follow-up di 2,96 milioni di anni-persona. Dopo aggiustamento multivariabile per i maggiori fattori di rischio comportamentali e dietetici, l'hazard ratio (Hr) della mortalità totale per l'aumento di una porzione al giorno di carne rossa non trasformata è stato di 1,13, e di 1,20 per quella trasformata. I valori corrispondenti per la mortalità cardiovascolare sono risultati 1,18 e 1,21, e quelli per la mortalità da cancro 1,10 e 1,16. Gli autori stimano che la sostituzione di 1 razione al giorno di altri cibi (pesce, pollame, nocciole, legumi, grano intero) al posto di una porzione al giorno di carne rossa si associ a una riduzione del rischio di mortalità dal 7% al 19%. Inoltre, si ritiene che, nel periodo osservato, il 9,3% delle morti tra gli uomini e il 7,6% dei decessi tra le donne possa essere prevenuto al termine se tutti gli individui avessero consumato meno di 0,5 razioni al giorno (circa 42 g/die) di carne rossa.
Arch Intern Med, 2012 Mar 12
Endocrinologia
Diabete negli ipertesi: meno danni cardiovascolari dai tiazidici
Nei soggetti ipertesi in terapia farmacologica, la comparsa di diabete correlato a un diuretico tiazidico determina minore impatto sugli eventi avversi cardiovascolari a lungo termine rispetto a quello determinato da altri antipertensivi (calcio-antagonisti e Ace-inibitori). È quanto emerso da un'estensione dello studio Allhat (Antihypertensive and Lipid-Lowering Treatment to Prevent Heart Attack Trial), coordinato da Joshua I. Barzilay del Kaiser Permanente of Georgia and Emory University School of Medicine, ad Atlanta (Usa). Sono stati selezionati 22.418 partecipanti allo studio principale, con diabete basale, diabete incidente (7,5% trattati con clortalidone, 5,6% con amlodipina, 4,3% con lisinopril), oppure senza diabete a un follow-up a 2 anni nel trial; tutti questi soggetti sono stati seguiti per un periodo medio di 6,9 anni tramite database nazionali. I partecipanti trattati con clortalidone affetti da diabete mellito - rispetto a quelli senza - hanno mostrato un rischio consistentemente minore, anche se non statisticamente significativo, di mortalità cardiovascolare, generale, non cardiovascolare e coronarica rispetto ai partecipanti in terapia con amlodipina o lisinopril con diabete incidente. In generale, i pazienti colpiti da diabete incidente hanno evidenziato un rischio coronarico superiore rispetto a quello dei soggetti senza diabete, rischio che in quelli trattati con clortalidone era significativamente inferiore rispetto a quelli in terapia con lisinopril.
Circ Cardiovasc Qual Outcomes, 2012 Mar 6.

Nefrologia
Più frequenti le apnee notturne, minore la funzione renale
Le apnee notturne sono frequenti nei pazienti con nefropatia cronica (Ckd), e aumentano con la riduzione della funzione renale. Quasi il 50% dei pazienti con Ckd e malattia renale allo stadio terminale (Esrd) sperimentano ipossia notturna, che può contribuire a perdita di funzionalità renale e ad aumento di rischio cardiovascolare. Il collegamento tra funzione respiratoria e renale è stato effettuato da un'équipe della university of Calgary (Canada) guidata da David D. M. Nicholl, che ha reclutato 254 pazienti da cliniche nefrologiche e unità di emodialisi per sottoporli a un monitoraggio notturno cardiopolmonare allo scopo di determinare la prevalenza di apnea notturna (indice di disturbo respiratorio =/>15) e ipossia notturna (saturazione d'ossigeno <90% per un monitoraggio =/>12%). I pazienti sono stati stratificati in 3 gruppi sulla base del tasso stimato di filtrazione glomerulare: eGfr =/>60 , Ckd (eGfr<60 non in dialisi) e Esrd (in emodialisi). La prevalenza di apnea notturna è risultata superiore nei pazienti con Ckd e Esrd.
Chest, 2012 Jan 5.
lunedì 5 marzo 2012
Antipertensivi: meglio se somministrati la sera
Antipertensivi: meglio se somministrati la sera
La modalità di somministrazione serale degli anti-ipertensivi sarebbe da consigliare almeno nei pazienti con insufficienza renale (IRC) e per i farmaci che agiscono sul sistema renina angiotensina (ACEI, Sartani). Questa è la conclusione cui perviene Hermida in uno studio che ha arruolato 661 pazienti con IRC. Lo studio, che ha però il limite di essere in aperto, ha valutato l'efficacia di una somministrazione di tutti gli antipertensivi al risveglio o di almeno un farmaco la sera, prima di andare a letto. È stata misurata la pressione delle 48 h basale, poi ogni 3 mesi o almeno ogni anno. Dopo un follow-up mediano di 5.4 anni, i pazienti che assumevano almeno 1 farmaco la sera avevano un rischio aggiustato per eventi cardiovascolari totali (infarti e angina, ictus, rivascolarizzazione, mortalità) ridotto di approssimativamente 1/3 rispetto ai pazienti che avevano assunto tutti i farmaci al mattino (p<0.001). Inoltre, i pazienti che assumevano i farmaci prima del riposo notturno avevano una pressione media notturna significativamente più bassa ed un migliore controllo della pressione nella rilevazione dinamica (p=0.003). Per ogni 5 mmHg di diminuzione della pressione sistolica notturna si è assistito a una riduzione del 14.5% del rischio di eventi cardiovascolari nel follow up (p<0.001). I migliori risultati si sono ottenuti però con ACEI e sartani; meno evidenti sembrano con i calcio-antagonisti (CCB) e nulli con i beta-bloccanti ed i diuretici. È possibile che per i beta-bloccanti sia importante la modulazione dell'attività simpatica (quindi meglio somministrarli al mattino) e per i diuretici il peggioramento del riposo notturno causato dell'inevitabile nicturia, se somministrati la sera. A nostro parere la novità del lavoro non consiste tanto nel fatto che si debba frazionare la somministrazione dei farmaci (in genere i nostri pazienti sono poli-trattati e da sempre si cerca di scaglionare le pillole) quanto nell'indicazione a somministrare gli ACEI e i sartani alla sera ed i calcio-antagonisti al mattino, mentre eravamo abituati a fare il contrario (per minimizzare i disturbi collegati alla possibile cefalea da CCB ed altri effetti collaterali).Hermida RC. J Am Soc Nephrol 2011; 22: 2313
Iscriviti a:
Post (Atom)